Critical review of AMR risks arising as a consequence of using biocides and certain heavy metals in food animal production: Introduction
Introduction to the report.
AMR is the resistance of a microorganism to an antimicrobial agent (a substance that kills or stops the growth of microorganisms) that was originally effective for treatment of infections caused by it, so that standard treatments become ineffective, and infections persist, increasing the risk of spread to others. In the context of clinical bacterial infections, resistance is most often defined based on likely clinical efficacy of an antimicrobial agent/bacteria combination; however clinical breakpoints (discriminatory antimicrobial concentrations used in the interpretation of results of susceptibility testing to define isolates as susceptible, intermediate, or resistant) are not available for all antimicrobial agent/bacteria combinations. This is particularly the case for agents such as biocides and heavy metals, where no internationally accepted breakpoints exist to define resistance. However, a further way of monitoring the development of AMR and any reduction in susceptibility or increase in tolerance is to use epidemiological cut-offs, which enables bacteria with reduced susceptibility to an antimicrobial agent to be distinguished from the wild type population and innate susceptibility. Within the literature, resistance is not always clearly defined, especially concerning studies investigating susceptibility/tolerance to biocides and heavy metals. For the purposes of this review the terms tolerance and reduced susceptibility are used when describing biocide and/or heavy metal “resistance”.
AMR is a complex issue driven by a variety of interconnected factors enabling microorganisms to withstand the killing or static effects of antimicrobial agents, such as antibiotics, antifungals, disinfectants, and preservatives. The widespread use of antimicrobial agents in all contexts is known to result in selection for AMR in microorganisms (O’Neill, 2016). There is also evidence that biocidal agents and/or heavy metals may, in some contexts, co-select for AMR in microorganisms (the focus of this review).
AMR and ARGs are a major public health issue worldwide and it is estimated that unless action is taken now to tackle AMR the global impact of AMR could be 10 million deaths annually from drug-resistant infections by 2050, costing up to US $100 trillion in health costs and cumulative lost economic output (O’Neill, 2016). Resistance to bacterial infections can make infections caused by these organisms difficult to treat and cause illness to persist, with recognised extra costs and increased morbidity and mortality (Likotrafiti et al., 2018).
Addressing the public health threat posed by AMR is a national strategic priority for the UK and led to the Government publishing both a 20-year vision of AMR and a 5-year (2019 to 2024) AMR National Action Plan (NAP) which sets out actions to slow the development and spread of AMR with a focus on antimicrobials. The NAP has adopted an integrated ‘One-Health’ approach which spans people, animals, agriculture, and the environment and calls for activities to “identify and assess the sources, pathways, and exposure risks” of AMR. The FSA have, and are continuing, to contribute to delivery of the 5-year NAP through furthering understanding of the role of the food chain and AMR, conserving the effectiveness of current treatments through the adoption of good hygiene practices, and encouraging the food industry to reduce usage of antimicrobials where possible. ARGs that result in resistance to what are termed ‘Critically Important Antimicrobial’s’ (CIAs) by the World Health Organisation (WHO) are of particular concern to the FSA.
AMR may be intrinsic or acquired by transfer mechanisms (Verraes et al., 2013). Transfer mechanisms include vertical gene transfer, acquired because of mutation (for example, genomic point mutations) [which in turn is passed on vertically], or the acquisition of ARGs within the same species or between different bacterial species by horizontal gene transfer [HGT] (Verraes et al., 2013; Munita & Arias, 2016). Bacteria may be resistant to just one antimicrobial agent or to several different agents (multi-resistant or multi-drug resistant (MDR) defined as resistance of a bacterial isolate to three or more classes of antimicrobials), with cross-resistance dependent on which ARGs and other mechanisms of resistance are present (such as, enzymatic, permeability barriers, and efflux pumps).
The transmission of antimicrobial-resistant microorganisms and ARGs to food and within the food chain is complex. Food can be contaminated with antimicrobial-resistant bacteria and/or ARGs in several ways (Verraes et al., 2013; Food Standards Agency, 2016) including (but not exclusively):
- Through contamination with antimicrobial-resistant bacteria in the environment.
- Through the presence of antimicrobial-resistant bacteria in food animals treated by antimicrobials during agricultural production.
- The possible presence of ARGs in bacteria that are intentionally added during the processing of food (starter cultures, probiotics, bio-conserving microorganisms, and bacteriophages).
- Through cross-contamination with antimicrobial-resistant bacteria and ARGs during food processing
Biocides
A biocide is defined as an active chemical molecule that controls the growth of, or kills, bacteria and other microorganisms in a biocidal product (SCENIHR, 2009; Wales & Davies, 2015; VKM, 2016). Biocidal substances act in different ways and sometimes several biocides are combined within a single product to increase the overall efficacy (VKM, 2016). The mechanisms of action and resistance/reduced susceptibility to a wide range of biocides on bacteria have been reviewed and described by McDonnell & Russell (1999), Ortega Morente et al. (2013), and Geueke (2014), amongst others. Many biocides act by effecting the plasma membrane of bacteria, because of which Gram-negative bacteria are generally less susceptible to many biocides than are Gram-positive bacteria (Denyer & Maillard, 2002; Wales & Davies, 2015).
Biocides are classified into different groups according to their application categories (Table 1). Biocides used in food animal production operations mainly act as disinfectants, sanitising agents, or antiseptics (SCENIHR, 2009; VKM, 2016; Donaghy et al., 2019). Examples of use include: the cleaning and disinfecting of buildings and equipment as well as decontaminating ponds and equipment in fish farming; in footbaths for operators outside animal housing; in livestock footbaths to treat and prevent the spread foot infections such as digital dermatitis; to clean udders of animals used for milk production; and for preserving specific products such as eggs or semen (SCENIHR, 2009; Wales & Davies, 2015; Donaghy et al., 2019; VKM, 2016). They may be used in anti-fouling paints used in aquaculture to reduce the growth of attached organisms on fish cages and nets (Burridge et al., 2010; Guardiola et al., 2012). Biocides are generally not used within body tissues (though some such as organic acids and essential oils (EOs) are added to animal feed and water as antimicrobial controls).
Table 1: Examples of biocidal products on the basis of chemical group (McDonnell & Russell, 1999; SCENIHR, 2009; VKM, 2016), not all example compounds listed may be permitted in the UK.
Chemical group | Example products/compounds | Examples of use |
---|---|---|
Antimicrobial dyes | Acridines, triphenylmethane dyes, quinones | Disinfection of equipment in fish farming. |
Aldehydes | Glutaraldehyde, formaldehyde, other aldehydes | Disinfection of equipment and environments in land farming and aquaculture |
Alcohols | Ethyl alcohol (ethanol), methyl alcohol (methanol), other alcohols | Antiseptics and disinfection agents. |
Biguanides | Chlorhexidine | General purpose disinfectant/antiseptic for cleansing wounds, skin, instruments, and equipment. Including as a dairy teat disinfectant. |
Chlorine compounds | Sodium hypochlorite (active agent in bleach), chlorine dioxide, electrolysed water. | Widely used for both antiseptic and disinfectant purposes in drinking water, wastewater, and in fish farms. |
Essential oils (EOs), plant compounds, and extracts | Menthol, tea tree oil, cinnamon oil, oregano oil, thyme | Disinfection, decontamination, including use in animal feed and water as antimicrobial controls. |
Iodine-releasing agents | Free iodine, iodophors | Used in teat dips for the prevention and control of mastitis in cattle. |
Organic and inorganic acids: esters and salts | Acetic acid (ethanoic acid), citric acid, lactic acid. | Disinfection, decontamination, including use in animal feed and water as antimicrobial controls. |
Peroxygens | Hydrogen peroxide, peracetic acid, ozone. | Disinfection, decontamination, and sterilisation, including the treatment of waste water and foods. |
Phenols | Creosols, non-coal tar phenols, halophenols, nitrophenols, bisphenols. | Antiseptics and disinfection agents. |
Quaternary ammonium compounds (QACs) | Benzalkonium chloride, cetrimide (alkyl trimethyl ammonium bromide, a mixture of three QACs). | General disinfection in the food industry. |
Biocidal products are regulated as they have the potential to cause harm to human health and/or the environment. Biocidal products are controlled in Great Britain (England, Scotland, and Wales) under the GB Biocidal Products Regulation (GB BPR) and in Northern Ireland under the EU Biocidal Products Regulation (EU BPR). A list of UK- authorised biocidal products is provided by the Health and Safety Executive (HSE).
This review has focused on the impact of only those biocides used in food animal production. For this reason, triclosan (5-chloro-2(2,4-dichlorophenoxy)phenol) was not considered, as this product was used almost exclusively in human related products, such as handwashes, toothpastes and other personal care products as well as being incorporated into other consumer products such as clothes. Due to health concerns and potential impact on the environment, it has been banned within the EU 27 and also in the USA. While increased AMR to this product is often discussed in the literature, it is of limited relevance to food animal production (Davies & Wales, 2019).
Heavy metals
Heavy metals are naturally occurring elements that have a high atomic weight and a density that is at least 5 times greater than that of water (Tchounwou et al., 2012). Some heavy metals (such as cobalt, copper, iron, manganese, molybdenum, selenium, and zinc) are essential in the diet of living things to maintain various physiological functions and are usually added as nutritional supplements in animal feed (Hejna et al., 2018). They have antimicrobial properties and may be used for this purpose in food animal production. In feed and as supplements they improve growth and prevent diseases via these antimicrobial properties by acting on the gut microbiota to reduce loss of nutrients and suppress gut bacteria, including pathogens (Li et al., 2022a). They may also be used as antimicrobials, The antimicrobial modes/mechanisms of action of heavy metals on bacteria /microbes have been reviewed by Lemire et al. (2013). Different metals cause discrete and distinct types of injuries to microbial cells as a result of oxidative stress, protein dysfunction, or membrane damage (Lemire et al., 2013). Due to the presence of toxic heavy metals in the general environment, many bacteria have evolved mechanisms of metal resistance (Vats et al., 2021). As discussed further, these mechanisms of resistance/tolerance may provide resistance/tolerance to other antimicrobials leading to co-selection for AMR.
Copper and zinc are used in the pig and poultry sectors as in-feed growth promotors and for enteric disease control (Wales & Davies, 2015). Zinc is used in aquaculture as a supplement in feed (Burridge et al., 2010; Yu et al., 2021). Heavy metals are often used in higher concentrations than needed to ensure adequate nutrition (Medardus et al., 2014; Yu et al., 2017). A survey of livestock feeds in England and Wales in 1999 reported concentrations of zinc and copper of 150–2,920 ppm and 18–217 ppm, respectively, in pig feeds and 28 to 4,030 ppm and 5 to 234 ppm, respectively, in poultry feeds (Nicholson et al., 1999). Since the bioavailability of metals in feed is usually quite low, unabsorbed heavy metals are excreted in faeces and may accumulate in soil, water, and sediments from agricultural practices. One study in the USA found 90% of in-feed copper and zinc fed to pigs was shed in faeces (Medardus et al., 2014). Although recently introduced new sources or forms of these metals with higher bioavailability allows for substantial reduction of dietary inclusion rates (Dębski, 2016). The total amounts and concentrations used of copper and zinc in feed may differ among countries, due to restrictions imposed by national legislation.
In two opinions, the EFSA Panel on Additives and Products or Substances used in Animal Feed (FEEDAP) recommended that zinc and copper contents be cut (EFSA FEEDAP Panel, 2014, 2016). They suggested that this would reduce residues of both of these metals in manure by 20%. Recommendations for zinc were based on animal requirements, while the opinion on copper in feed also considered its impact on AMR. These recommendations have since been enacted in EU legislation. Permitted maximum zinc contents animal feed in the EU (Regulation 2016/1095) are: 180 mg zinc/kg for salmonids and in milk replacers for calves; 150 mg zinc/kg for piglets, sows, and all fish species other than salmonids; and 120 mg zinc/kg for other species. Permitted maximum copper contents animal feed in the EU (Regulation 2018/1039) are: 15 mg copper/kg for bovines (cattle) before the start of rumination; 30 mg copper/kg for other bovines (cattle); 15 mg copper/kg for ovines (sheep); 15 mg copper/kg for caprines (goats); 150 mg copper/kg for piglets suckling and weaned up to 4 weeks after weaning; 100 mg copper/kg for piglets from 5th week after weaning up to 8 weeks after weaning; 50 mg copper/kg for crustaceans; and 25 mg copper/kg for other species.
Yu et al. (2017) theorised that certain forms of heavy metals (as stable metal compounds that do not release free metal ions) may provide nutrition to food-producing animals but not be toxic to bacteria, and hence their use in feed would not co-select for resistance in bacteria (Yu et al., 2017). There does not appear to be any evidence supporting this hypothesis.
Other uses of heavy metals include use in livestock footbaths to treat and prevent the spread foot infections such as digital dermatitis (Bell et al., 2014; Yu et al., 2017) and wound dressings (Wales & Davies, 2015). As discussed in more detail later in this report, following concerns over therapeutic use of zinc in animal production potentially leading to an increased prevalence of livestock associated methicillin-resistant Staphylococcus aureus (LA-MRSA) zinc is now only permitted in the EU and UK at concentrations up to 150 ppm for nutritional use (Veterinary Medicines Directorate, 2022). Copper is the principal biocidal component of anti-fouling paints used in aquaculture to reduce the growth of attached organisms on fish cages and nets (Burridge et al., 2010; Guardiola et al., 2012). Copper has also been studied as a possible antimicrobial alternative to stainless steel surfaces in food production and processing (Pontin et al., 2021). The use of silver and zinc nanoparticles as antimicrobial controls for a wide range of applications, including in food animal production, have received considerable attention in recent years (McDonnell & Russell, 1999; Maillard & Hartemann, 2012) and were included in this review. A list of heavy metals considered in this review is shown in Table 2.
Table 2: Heavy metals that were considered or excluded from this review.
Essential metals (Authorised in animal feed and drugs or * antimicrobial control) |
Non-essential metals (Considered contaminants/ undesirable substances), excluded from review |
---|---|
Cobalt (Co) Copper (Cu) Chromium (Cr) Iron (Fe) Manganese (Mn) Molybdenum (Mo) Selenium (Se) Silver (Ag)* Zinc (Zn) |
Arsenic (As) Cadmium (Cd) Mercury (Hg) Lead (Pb) |
Other metals (cadmium, lead, mercury) have no established biological functions and are considered as contaminants/undesirable substances (Hejna et al., 2018). As requested in the FSA specification they were not reviewed in this study. Arsenic has been intentionally used in animal feeds and drugs (to reduce coccidial infection and promote growth) in the past (Silbergeld & Nachman, 2008; Rensing et al., 2018) but it is now banned in most countries, including the UK, the EU 27, and USA, due to concerns on its potential to cause harm to human health and to the environment and was not included in this review.
Mechanisms of action of biocides and heavy metals and similarities in resistance to antimicrobials
Since biocides and their applications are diverse, so are the mechanisms of action of different biocides on bacteria, and consequently on antimicrobial-resistant bacteria and their genes. Biocides have been used extensively in a wide range of applications to control and kill bacteria (Jones & Joshi, 2021). Furthermore, the same biocide can be used for different applications, as the action is concentration dependent and most bacteriostatic and bactericidal actions depend on the concentration used. There are multiple mechanisms of action involving different targets that have been described and they can (i) interfere with the replication of nucleic acids, (ii) interfere with protein synthesis, (iii) alter the structure and function of cell wall, (iv) increase permeability and disrupt the cytoplasmic membrane, and (v) inhibit intermediate metabolic pathways (Liwa & Jaka, 2015).
Quaternary Ammonium Compounds (QAC)s, alcohols, and biguanides lead to bacterial cell lysis with their mode of action involving interactions with bacterial outer membranes and with the cytoplasmic membrane which causes loss of integrity and leakage of the intercellular components and lysis (Jones & Joshi, 2021). Another group of biocides (protonophores) which include the weak acids, such as citric acid and benzoic acid, interfere with the pH balance of cells causing cytoplasm acidification and disruption of the proton-motive force (PMF). This interferes with the metabolic generation of energy and the cell starts to die. Oxidising biocides (such as hydrogen peroxide and ozone) have a rapid killing action by oxidising organic materials by releasing free radicals or by halogenating molecules within the cell. Other electrophilic biocides (such as glutaraldehyde and formaldehyde) cause enzyme inactivation by interaction with cellular components by covalent linkage (Amjad, 2010). The heavy metals, silver and copper, also have this type of mechanism. Mechanisms of antimicrobial action of and resistance/reduced susceptibility to silver have been described by Maillard & Hartemann (2012).
As discussed further in this report, the widespread use of biocide products in diverse applications often used at inappropriate concentrations has been of some concern since such usage may cause development of AMR in some bacteria (Wesgate et al., 2016).
Heavy metals may be toxic to bacteria. As previously mentioned, several possible modes of antimicrobial action of heavy metals have been reported (Lemire et al., 2013). These are: (i) protein dysfunction, (ii) production of reactive oxygen species (ROS) and antioxidant depletion, (iii) impaired membrane function, (iv) interference with nutrient uptake, and (v) genotoxicity. These mechanisms have been reviewed by Lemire et al. (2013).
The following factors influence the efficacy of antimicrobial agents and the resistance/tolerance of bacteria to such agents (whether biocides and/or heavy metal) (SCENIHR, 2009; Wales & Davies, 2015; VKM, 2016):
- Innate or intrinsic resistance of bacteria, such as the presence of, or accessibility to the target of the agent.
- Number and location of bacteria.
- Age of bacterial community.
- State: vegetative cells or spores.
- Concentration and potency of the antimicrobial agent (concentrations below the minimum inhibitory concentration (MIC) may co-select AMR).
- Physical and chemical factors (for example, pH, temperature, salt, mode of application/contact).
- Organic and inorganic materials.
- Duration of exposure (time).
- Attachment of bacteria and presence and state of biofilms.
The influence of these factors on resistance/tolerance will be discussed in further sections of this report.
Many bacteria have evolved mechanisms of resistance to toxic agents. These mechanisms of resistance may provide resistance to other antimicrobials, thereby leading to co-selection for AMR. There are several similarities and differences between antibiotic and biocide/heavy metal resistance/tolerance as shown in Table 3.
Table 3: Similarities and differences between antibiotic and biocide/heavy metal resistance/tolerance (adapted from Weber et al., 2019).
Similarities | Intrinsic resistance (for example, spores are resistant to alcohols) and extrinsic resistance (for example, efflux pumps for heavy metals) are well described. Acquired mechanisms of resistance are similar (for example, impermeability, efflux pumps). Biofilms impair inactivation/killing. Inactivation is dependent on the concentration and duration of contact with the antibiotic, biocide, or heavy metal. |
---|---|
Differences | Most antibiotics inhibit a specific target in a biosynthetic process. Most biocides have multiple concentration-dependent targets, with subtle effects occurring at low concentrations and more damaging ones at higher concentrations. |
Horizontal Gene Transfer (HGT) of resistance genes
Resistance genes (whether ARGs or biocide resistance genes (BRGs) or heavy metal resistance genes [HMRGs]) in bacteria can be transferred to other bacteria through Horizontal Gene Transfer (HGT). Thus, commensal non-pathogenic bacteria with resistance genes can act as a reservoir for ARGs, BRGs, and HMRGs and transfer resistance to non-resistant human pathogenic bacteria (Bengtsson-Palme, 2017). HGT is driven by mobile genetic elements (MGEs), such as plasmids, integrons, transposons, and staphylococcal cassette chromosome elements that facilitate the movement, transfer, and integration of genes between cells (Bennett, 2008). Resistance genes are not always associated with cultivable ‘live’ bacteria (Figure 1). Viable but non-culturable bacteria (VBNC) may express genes after “lethal” treatments (James et al., 2021). Non-cellular ARGs, which covers genes encapsulated in membrane vesicles (MVs), bacteriophages, or gene transfer agents (GTAs), can persist after disinfection, and can transfer to recipient bacteria in the absence of a live donor bacteria (Woegerbauer et al., 2020; James et al., 2021). The frequency of HGT largely depends on the properties of the MGEs, MVs, or bacteriophages, the characteristics of the donor and recipient populations, and the environment (Verraes et al., 2013; Rossi et al., 2014). As will be discussed in further sections of this report biocides and heavy metals may influence HGT.
Figure 1: Forms and origins of resistance genes quantified by molecular biology approaches.
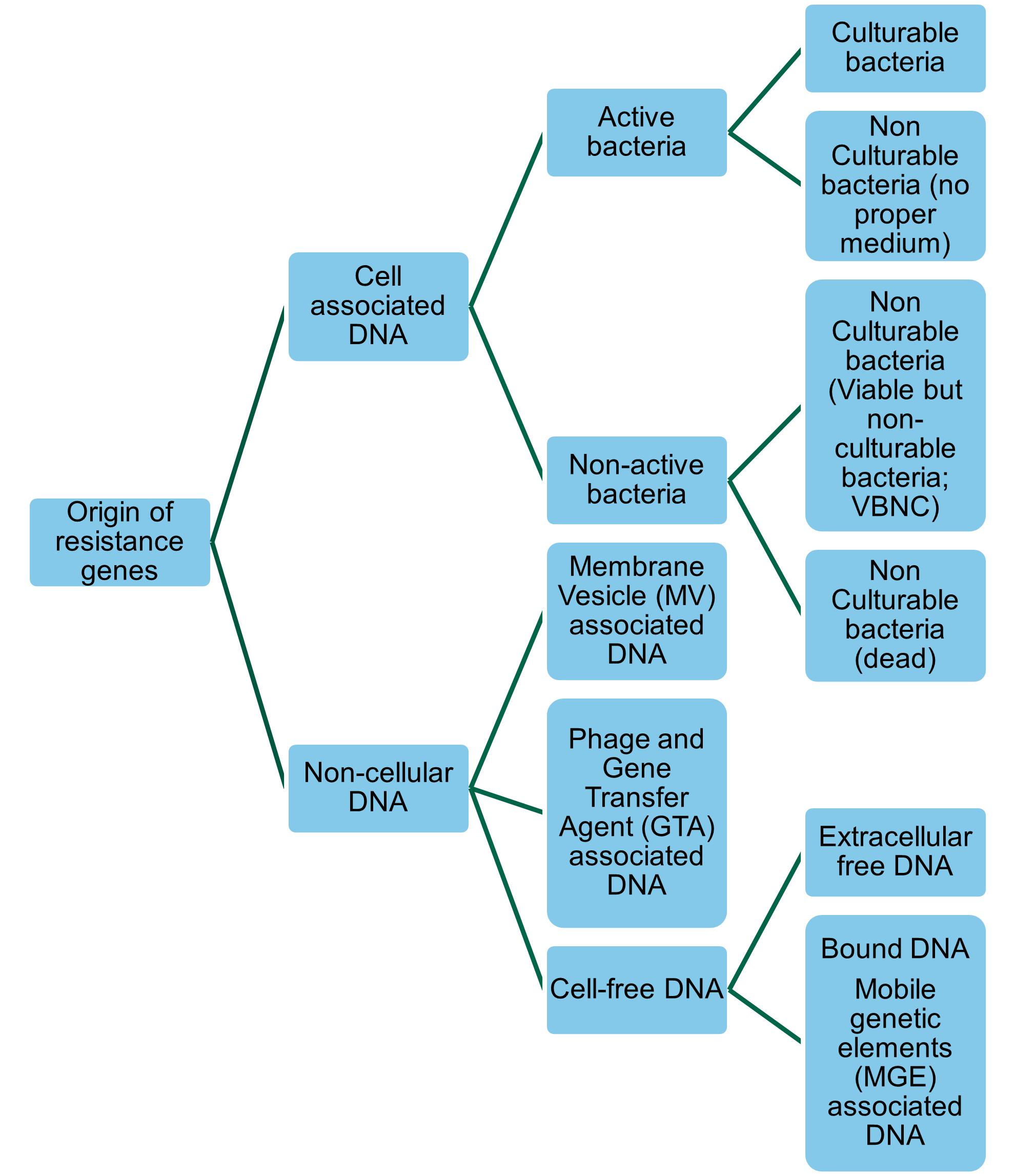
A more detailed discussion of HGT mechanisms can be found in section 13 of this report.
Role of biocides and/or heavy metals in co-selecting AMR
Co-selection mechanisms for biocides and/or heavy metals and clinically- as well as veterinary-relevant antibiotics have been described by Seiler & Berendonk (2012), Wales & Davies (2015), Donaghy et al. (2019), Davies & Wales, (2019), Cheng et al. (2019), and EFSA BIOHAZ Panel (2021), amongst others. There are two main types of related resistance co-selection mechanisms:
- Cross-resistance – where resistance is due to physiological adaptations that provide resistance to a number of toxic agents (such as biocides and antibiotics), examples being efflux pump upregulation, over expression, or reduced cell wall/membrane permeability.
- Co-resistance/co-transfer – where resistance to different toxic agents is dissimilar but there is a genetic link between resistance to different agents, such as the co-location of different resistance genes on the same MGE (mobile genetic elements), such as plasmids but also on chromosomes. Because of the genetic linkage between such resistance, exposure to any of these groups of antimicrobials, or any combination of them, could co-select for the maintenance of the whole MGE and all its associated resistance phenotypes.
Thus, there are some phenomena that confer reduced susceptibility both to antibiotics and to biocides and/or heavy metals (Wales & Davies, 2015; Donaghy et al., 2019; Cheng et al., 2019). These phenomena may be normally present (intrinsic) in the bacteria, or readily acquired by mutation or genetic transfer under appropriate conditions (Wales & Davies, 2015; Donaghy et al., 2019). Phenomena such as spore formation, biofilm formation, nutrient stress responses, low cell wall permeability, and efflux pumps (transport proteins involved in the extrusion of toxic substrates from within cells into the external environment [Webber & Piddock, 2003]) are resistance mechanisms that may enable bacteria to resist antibiotics, biocides, and/or heavy metals (Wales & Davies, 2015; Donaghy et al., 2019). Efflux pumps may expel a broad range of unrelated and structurally diverse compounds including antibiotics, biocides, and/or heavy metals. Thus, whether intrinsic or acquired, bacteria possessing efflux pumps have substantial potential for cross-resistance to antibiotics, biocides, and/or heavy metals, though this does depend on the nature of the efflux pump (Webber & Piddock, 2003; Wales & Davies, 2015).
Resistance may be acquired through the release of resistance genes in MGEs. They may potentially allow some proportion of the bacterial population to survive an otherwise terminal challenge, increasing the risk of selection of organisms permanently adapted to the antimicrobial agent (Wales & Davies, 2015). There can be a genetic link between resistance to different agents (co-resistance) through the co-location of resistance genes on MGEs (Bloomfield, 2002; Wales & Davies, 2015; Ciric et al., 2011).
Resistance in many antimicrobial-resistant bacteria is encoded by genes that are carried on large conjugative plasmids. These plasmids typically contain multiple ARGs as well as genes that confer reduced susceptibility/tolerance to biocides and/or heavy metals (Gulberg et al., 2014). An example of co-resistance are class 1 integrons, which encode a QAC efflux mechanism (qacEΔ1) plus sulphonamide resistance (sul1) and variable other ARGs (Carattoli et al., 2001). The co-existence of blaCTX-M (an ARG encoding resistance to 3rd generation cephalosporins, critically important antimicrobials [CIAs]) and oqzAB (an efflux pump mediating MDR) and pco and sil operons (encoding copper and silver tolerance, respectively) have been reported on the same plasmid isolated from E. coli in food-producing animals (Fang et al., 2016; Zingali et al., 2020). The co-existence of heavy metal tolerance operons (including silA, encoding for tolerance to silver) in plasmids harbouring ARGs including blaCTX-M-2 and the quinolone resistance gene, qnrB isolated from Salmonella spp. from Brazilian poultry has been observed (Ferreira et al., 2019; Galetti et al., 2021). Plasmids isolated from E. fergusonii from poultry have been observed to harbour ARGs and heavy metal tolerance operons (Galetti et al., 2019).
An analysis of the co-occurrence of resistance/tolerance genes to antibiotics, biocides, and metals by Pal et al. (2015) concluded that plasmids provide limited opportunities for biocides and metals to promote HGT of AMR through co-selection (though this was more common in bacteria of animal origin), whereas greater possibilities exist for indirect selection (and therefore clonal selection via chromosomal BRGs and HMRGs).
There is evidence that zinc and/or copper may co-select for LA-MRSA due to co-location of the zinc/copper HMRG czrC and the methicillin resistance gene mecA within the staphylococcal cassette chromosome (SCC) SCCmec element (Aarestrup et al., 2010; Cavaco et al., 2010; Xue et al., 2015; Argudín et al., 2016; Hau et al., 2017; Poole et al., 2017; Jensen et al., 2018). SCCmec is a MGE that carries the mecA gene (or its homologue mecC encoding resistance to methicillin and all β-lactam drugs) and other functional genes (including HMRGs), and can transfer to other Staphylococcus spp.
The persistence of bacteria in food production environments is often associated with their biofilm forming ability. Biofilms are complex structures formed by different or single types of bacteria adhering to surfaces which may enhance resistance to different antimicrobial agents (Uruén et al., 2021). Biofilms have an extracellular matrix that provides a diffusion barrier and an enhanced medium for bacterial signalling and genetic exchange, plus a potential site for neutralisation or binding of chemical agents and an extracellular site for sequestration of metal ions (Wales & Davies, 2015; Donaghy et al., 2019). Once a biofilm forms, bacteria become more resistant to external factors. Bacterial biofilms have been well documented to be highly resistant to antimicrobials, whether biocides or antibiotics (Maillard, 2020). The presence of multiple species may allow for HGT of resistance genes between different bacteria (Allen et al., 2016). Biofilms can generate a state of hypermutability (capability for excessive mutation) in part due to stress and slower growth that stimulates the development of resistance which may co-select for AMR (Yu et al., 2017; Uruén et al., 2021).
There is evidence that some adaptations that enable resistance to antimicrobial agents may result in associated costs to the organism, usually termed “fitness cost”. An example is broad substrate efflux pumps, which consume cell energy resources and indiscriminately remove some useful metabolic substances from the cell (Wales & Davies, 2015; Davies & Wales, 2019). Plasmids encoding resistance to biocides or heavy metals plus antibiotics have been cited as another example (Gulberg et al., 2014). It has been reported that compensatory mutations can arise which offset such plasmid fitness costs (Hall et al., 2021). This has been reported for Pseudomonas fluorescens when acquiring a conjugative plasmid which encodes tolerance to mercury with acquisition resulting in the formation of small colony variants. After repeat passage transconjugants (bacteria that had incorporated DNA from others via conjugation) resumed normal colony size, which was linked to chromosomal mutation (Hall et al, 2019).
A further mechanism that may be relevant to co-selection is the influence of biocides and/or heavy metals on gene transfer (Wales & Davies, 2015; Davies & Wales, 2019). There is some evidence that while some biocides at sub-inhibitory concentrations may inhibit gene transfer, others may increase the efficiency of gene transfer.
Maertens et al. (2019) observed that sub-inhibitory concentrations of a QAC (benzalkonium chloride) had no effect on the conjunctive transfer of ARGs in E. coli originating from poultry. While sub-inhibitory concentrations of cetrimide (alkyltrimethylammonium bromide, a mixture of three QACs) have been observed to increase the transduction of plasmid pWG613 via a bacteriophage in S. aureus (Pearce et al., 1999). Likewise, sub-inhibitory concentrations of chlorhexidine (24.4 μg/L), gentamicin (0.1 mg/L) and sulphamethoxazole (1 mg/L) have been observed to significantly increase the frequencies of transfer of antibiotic resistance in E. coli by conjunction, while other biocides had no effect (Jutkina et al., 2018). Experiments involving field (sewage) bacterial communities of E. coli showed that the efficiency of conjugative transfer between genera may be enhanced in the presence of sub-inhibitory concentrations of biocides, namely free chlorine (0.1−1 mg/L), chloramine (0.1−1 mg/L), and hydrogen peroxide (0.24−3 mg/L) (Zhang et al., 2017a).
These studies suggest that the persistence of low concentrations of some biocides in the environment may accelerate the transfer of ARGs. Persistence will depend on the nature of the biocide. In all cases exposure to biocide concentrations higher than the MIC significantly suppressed transfer of ARGs. Heavy metals, such as copper and zinc, have been reported to facilitate HGT of ARGs in water (Zhang et al., 2018; Cheng et al., 2019; Wang et al., 2020). Whether they do in other environments appears not to have been studied. Our literature search has not identified any studies that have specifically looked at the effect of sub-inhibitory concentrations of biocides on gene transfer under the field conditions present in food animal production. Many studies appear to report an association or correlation in BRGs and/or HMRGs and ARGs due to no clear evidence of co-selection mechanisms.
Davies & Wales (2019) have postulated that, given that there is evidence that low concentrations of antimicrobials, whether antibiotics or biocides, elevate the rate of random mutations in exposed bacterial populations (Cogliani et al., 2011) resulting in spontaneous mutants showing cross-resistance to biocides and antibiotics. According to Maillard (2020), mutations resulting from biocide exposure have mainly been investigated with triclosan, but some studies have looked at other biocides, such as QACs. A laboratory study observed that one single exposure to the working concentration of certain biocides (one a mixture of aldehydes and QACs, one a halogenated tertiary amine compound) may provoke the selection of mutant Salmonella Typhimurium with an efflux mediated multidrug resistance (Whitehead et al., 2011).
Role of concentration of biocides and/or heavy metals in co-selecting AMR
For selection of biocide-resistant bacterial strains to occur, some proportion of the population would be expected to survive the application of biocides. The mode of use of biocides would therefore appear to offer fewer opportunities for survivor selection (Wales & Davies, 2015; Donaghy et al., 2019), compared with heavy metals. Biocides are intended to be lethal/inhibitory, usually after a single application, so are used in the field at concentrations that are higher than the MIC determined in the laboratory. In addition, the Minimum Bactericidal Concentration (MBC) may be determined, which is the lowest concentration of an antimicrobial agent required to kill 99.9% of bacteria over a fixed, somewhat extended period, such as 18 hours or 24 hours, under a specific set of conditions. Whereas the MIC test demonstrates the lowest level of antimicrobial agent that prevents growth, the MBC demonstrates the lowest level of antimicrobial agent resulting in microbial death. The use of biocides in the presence of heavy organic soiling or with diluting water containing interfering organic or mineral substances may produce marked reductions in efficacy even at recommended application concentrations. This may occur on farms or in aquaculture and may reduce effective inhibitory concentrations to sub-inhibitory in practice (Wales & Davies, 2015). For example, the efficacy of the use of biocides where heavy soiling is present, such as their use on vehicle wheels and undercarriages, has been questioned by Maillard (2018). Furthermore, some biocides (such as organic acids and EOs) may be used in practice at sub-inhibitory concentrations (below MICs) in feed and water as growth promoters and for pathogen control (Wales & Davies, 2015).
Low concentrations of antimicrobials in the environment may provide resistant strains of bacteria with a competitive advantage since they may be able to grow in such environments faster than non-resistant strains. The minimum selective concentration (MSC) has been defined as the lowest concentration of an antimicrobial at which resistance is positively selected or co-selected. As highlighted by FAO/WHO, 2019 there are little data on what these threshold values should be in order to inform suitable standards for biocide and metal concentrations in food animal production. There is evidence that the MSC is affected where species of bacteria are embedded within complex communities, such as animal faeces, and may be higher than single strain based estimates (Klümper et al., 2019). FAO/WHO (2019) note that the body of evidence to establish such thresholds is likely to take a considerable time to accumulate. Some studies which have been carried out on MSCs for heavy metals in manure, slurry, and soil are discussed later.
In two reviews Kampf examined published evidence on the cross-resistance of Gram-positive (Kampf, 2018) and Gram-negative (Kampf, 2019) bacterial species to biocides. He concluded that there is evidence that sub-inhibitory concentrations of benzalkonium chloride (a QAC used as a sanitiser) and chlorhexidine (a biguanide used as an antiseptic and disinfectant) may co-select for AMR in both Gram-positive and Gram-negative bacteria. There was evidence for sodium hypochlorite cross-resistance in Gram-negative species, but not Gram-positive. In contrast there is no evidence that cross-resistance to antibiotics has been described after low level exposure to glutaraldehyde, ethanol, propanol, peracetic acid, povidone iodine, and polyhexanide in Gram-positive and Gram-negative bacteria species. Studies such as Molina-González et al. (2014) have reported that sub-inhibitory concentrations of trisodium phosphate, sodium nitrite, and sodium hypochlorite may result in increased AMR in strains of S. enterica. Other studies (Soumet et al., 2012) have observed that sub-inhibitory concentrations of other QACs (didecyl dimethyl ammonium chloride (DDAC) and dioctyl dimethyl ammonium chloride (OCDAC)) may co-select for AMR. While Thomas et al. (2000) observed, in a laboratory study, that repeated exposure to sub-inhibitory concentrations of chlorhexidine created stable resistance in Pseudomonas aeruginosa, there was no cross-resistance to any antibiotics tested, although there was some resistance to benzalkonium chloride.
Alternatively, there is the possibility that intact BRGs could survive the application of biocides on surfaces via MGE and transfer to other bacteria via HGT. There appears to be little literature that considers this possibility. In addition, such exposure could trigger a SOS response in bacteria (a response to DNA damage) and this response has been associated with generation of genetic diversity with AMR variants through the formation of reactive oxygen species and mutagenesis. Whether exposure to some biocides can lead to specific or multiple resistances to antibiotics (including CIAs) used in clinical settings remains to be elucidated. The activation of the SOS response has been found to be associated with the formation of biofilms perhaps due to the slower growth rates involved although antibiotic resistant variants may emerge. A further consideration is that the SOS response is important for prophage activation. Activation of the SOS response could therefore result in the release of phage particles which many carry ARGs and thereby contribute to HGT (Diard et al., 2017). There appears to be little literature that considers any of these possibilities.
Unlike biocides, heavy metals are often used at sub-inhibitory concentrations providing more potential for resistance and co-selection of AMR to emerge (Wales & Davies, 2015).
Aims/objectives of this study
The review question was:
“Do biocides and/or heavy metals used in food animal production have an impact on the development of AMR in the food chain?”
The review was structured and aimed at addressing the following key questions (terms of reference) provided by the FSA:
- Is there evidence in the literature to show that biocides and/or heavy metals used in food animal production have an impact on the development of AMR?
- How long are biocides and/or heavy metals (used in food animal production) able to persist in animal production environments and how does this impact on the development of AMR and associated risks?
- What evidence from the literature is there that biocide and/or heavy metal associated AMR enters the food chain through products of animal origin or as a result of crop contamination?
- Is there a potential risk to the consumer from AMR acquired through the use of biocides and/or heavy metals in food animal production?
A central question was whether the release of biocides (in particular disinfectants) and/or heavy metals from food animal production has the potential to create local concentrations where AMR can emerge and spread (as bacteria or genes) and whether this presents a potential risk to the consumer as a result.