The Future of Animal Feed
Future of protein sources in livestock feeds: implications for sustainability and food safety
The feed-food competition for environmental and economic resources raises increasing concerns about the production and supply of protein for the global livestock sector.
Risks to food-security and approaching deadlines for global sustainable development, means exploring the potential for alternative protein feeds is imperative. However, as the use of alternative feeds for livestock production is still at its infancy, it is critical that potential direct or indirect food safety risks are evaluated before implementation at commercial scales. This Rapid Evidence Assessment (REA) offers a lens that focuses on the potential opportunities and threats of such alternatives for the sustainability and food safety of the global livestock sector.
Four potential alternative protein sources for livestock feeds are identified and evaluated through this REA:
- genetically modified / engineered protein crops and alternative cultivation methods
- cellular agriculture
- former foods, food waste and industry by-products and waste streams
- animal by-products and insects
Through this analysis, a strategic policy roadmap and research agenda are synthesised to facilitate higher-level policy making, supporting local solutions for global sustainable development and a more food-secure future. The four broad directions for policy making and research the REA proposes are:
- decoupling protein production from fossil fuel
- developing sustainable economic strategies for alternative proteins at a subnational level
- supporting circular livestock feed solutions
- further enhancing the feed and food regulatory system
Background
The future of livestock feed is of major concern to stakeholders of the agri-food industry, including the producers and consumers of livestock products, and regulatory authorities that need to monitor the sustainable development of the sector and ensure food safety [Makkar, 2018; Gurgel, Reilly & Blanc, 2021]. Feed production is the largest contributor to environmental and economic impacts associated with livestock production systems. It is predicted that an increased demand for animal-sourced food will lead to an almost twofold increase in global livestock production by 2050 [FAO, 2019]. The majority of livestock production systems globally rely heavily on unsustainable, plant-based sources to cover the needs for protein in livestock nutrition [FAO, 2018]. Feed production competes directly for resources required to produce human food, particularly due to the changing climate. Soybean, sunflower, and rapeseed meals, the most popular conventional plant-based protein sources in feed formulations, are directly linked to negative impacts including atmospheric pollution and global warming, acidification and eutrophication, deforestation and land degradation impacts [LEAP, 2017; Andretta et al., 2021]. These environmental impacts cause significant negative secondary effects on habitat conservation and flora and fauna biodiversity, and public health [Semper-Pascual et al., 2019; Adam et al., 2021].
Considering the planet’s limited biophysical capacity, and instabilities in macroeconomic, geopolitical, and socioeconomic factors, investigating more resilient and sustainable feed ingredients, hence called alternative, is critical to improving the sustainability of the livestock sector and meeting the increasing requirements both for livestock and human nutrition [van Huis & Oonincx , 2017; van Hal et al., 2019; Te Pas et al., 2021]. In doing so, it is important to consider potential impacts associated with such alternatives with regard to the environment, economy, and society, to ensure effectiveness, viability, and sustainability of livestock feed, as well as ensure food safety and prevent threats to human health [Muscat et al., 2020]. Using insect meals as a case in point, Box 1 presents examples of benefits and risks associated with the implementation of this alternative protein source, and highlights the complexity in identifying optimal, sustainable and safe solutions.
Objectives
This Rapid Evidence Assessment (REA) report highlights the opportunities and limitations of existing solutions for sustainable and safe protein in livestock feeds and proposes scenarios and directions for future biotechnological developments for the production of alternative protein sources. By doing so, the REA offers a research and policy roadmap to facilitate Food and Standards Agency (FSA) strategic development towards achieving a more sustainable and safe livestock feed sector, targeting global Sustainable Development Goals (SDGs) and ensuring food safety. The specific objectives of this report are:
- To identify alternative sources for protein feed ingredients and discuss their potential to substitute conventional protein crops in future livestock feed formulations.
- To evaluate the specific sustainability and food safety associated opportunities and risks they present, as well as potential trade-offs.
- To identify key relevant policy recommendations to inform FSA’s anticipatory strategic and regulatory policy when considering the incorporation of alternative protein ingredients for sustainable and safe livestock feeds.
Research questions
The report attempts to address the following specific research questions:
- What are some alternative protein sources that could substitute conventional, unsustainable protein feeds?
- How environmentally friendly, commercially viable, affordable, and safe are they likely to be?
- Do they pose any significant risks to feed and food safety and security?
- How can they contribute towards sustainable development of the livestock sector?
Methodology
The report adopts a holistic approach to review and evaluate sustainability implications of potential alternative protein feed ingredients, considering equally the environmental, economic and social dimensions throughout. This report was based on a rapid evidence assessment and synthesis of available scientific and grey literature, including peer-reviewed scientific articles, news articles, industry and government reports and relevant databases of the agri-food sector (e.g., Farm Accountancy Data Network, 2022; Tables of composition and nutritional values of feed materials, 2004). The research method involved extensive desk-based, qualitative research. The report adopts a holistic approach to review and evaluate sustainability implications of potential alternative protein feed ingredients, considering equally the environmental, economic and social dimensions throughout.
Structure of the report
The following sections of the report present a brief description and overview of potential alternative protein sources that can be used to substitute conventional protein crops in livestock feeds. Specifically, Chapter 2 identifies categories of alternative protein sources and drivers that call for a shift from conventional protein production. The categories are identified through extensive scientific and grey literature review that focused on alternative protein sources whose implementation on small scales had been previously explored, and whose upscale may be enabled by the technological advancements of the next 10 years. The assessment discusses the aspects of production and supply economics, and relevant economic uncertainties that may affect the implementation of alternative proteins in future livestock feeds. Such issues must be considered as they often largely dictate stakeholder investment behaviour and willingness to adopt alternative strategies in their production systems Through this analysis, the REA attempts to present pragmatic solutions considering a 10-year horizon while acknowledging the potential existence of further alternatives that may be currently under development.
They specific four categories presented in this REA are:
- Protein from genetically modified / engineered protein crops and alternative protein crop growing methods,
- Protein from cellular agriculture,
- Protein from former foods, food waste and industry by-products and waste streams, and
- Protein from animal by-products and insects.
For each of these categories, Chapters 3 through 6 present an assessment of the potential opportunities for improvement of livestock feed sustainability and of the potential risks to sustainability, considering the environmental, economic and social dimensions. Based on this assessment, Chapter 7 identifies key trade-offs within and across the three pillars of sustainability – environment, economy, society – and the state-of-the-art in trade-off assessment methods. Chapter 8 then discusses emerging threats for sustainability of the livestock feed production sector within and beyond a 10-year time horizon. Finally, Chapter 9 concludes the report by synthesising key policy pathways to facilitate the development of strategic and regulatory plans for FSA towards future sustainable livestock feed formulations.
Environmental drivers
Livestock feed is the largest user of available agricultural land globally covering approximately two times more land than food crop production, with the increase in soybean cultivations being a primary driver for land-use change (Fig 1.-2.) [Manceron et al., 2014]. While technological advances and improved management practices in livestock production have driven a reduction in pasture land-use over the past two decades, global cropland for livestock feed has exhibited an increasing expansion particularly in the global South [Winkler et al., 2021]. The expansion of soy production for livestock feed has been associated with increased land degradation and land-use related impacts (i.e., deforestation) in one of the most important biodiversity hotspots globally, the Brazilian tropical savanna (Cerrado) (Fig.2) [Song et al., 2021]. While, strict policies have been implemented to mitigate severe environmental impacts of farming expansion, such as deforestation (e.g., the “Soybean Moratorium”) [Kastens et al., 2017], this had limited effect in reducing the intensity of resource use including land [Lathuilliere et al., 2017].
Figure 1: Expansion of the share of conventional protein crops in livestock feed formulations between 1961-2009 based on FAOSTAT. Source: Manceron et al. (2014)
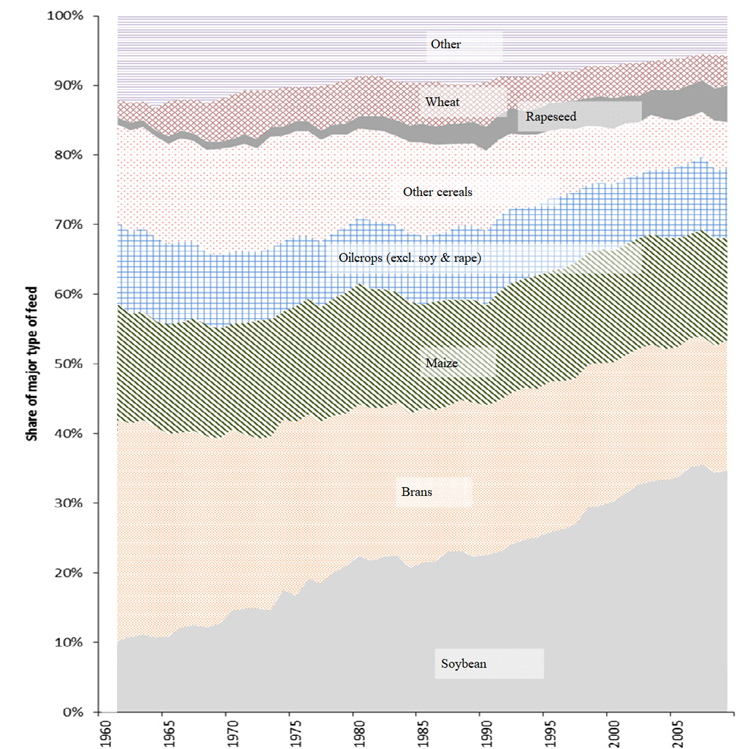
Figure 2: Land-use impacts by soybean crop expansion in South America presented through overlays of annual soybean classification maps from 2001 to 2019. Source: Song et al. (2021)
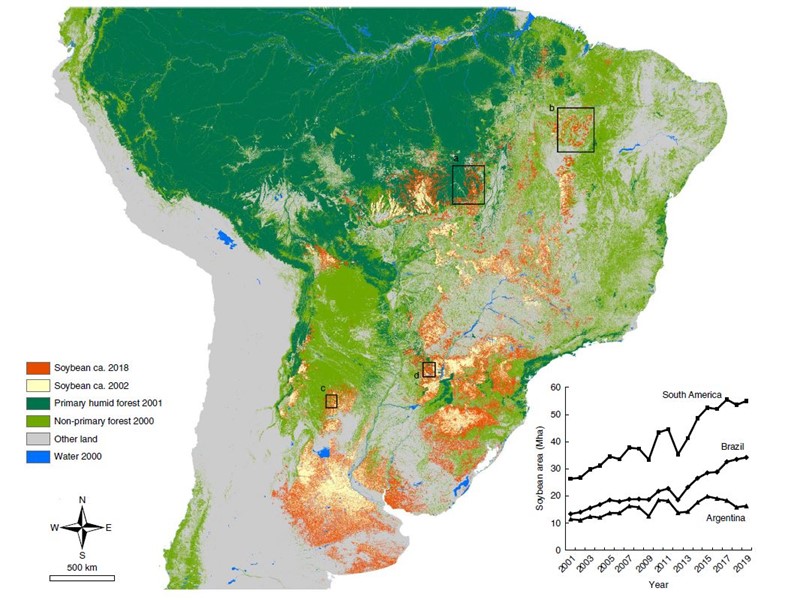
Many of the land-use related impacts discussed previously are also associated with greenhouse gas emissions (GHGs) arising from protein crop production [Castanheira & Freire, 2013]. Soil management practices affect the release of CO2 from the land and into the air, and therefore adopting alternatives that help reduce the need to disturb the soils can positively contribute in the reduction of anthropogenic GHGs [Johnson, 2018]. Furthermore, the expansion of the protein crop sector in the past two decades has led to a global increase in biomass burning, a practice used for deforestation, largely contributing to anthropogenic climate change and atmospheric pollution [Hoang & Kanemoto, 2021]. In addition to slash-and-burn deforestation, the burning of agricultural wasted biomass from conventional protein crops has worsened issues of smoke haze and airborne particulate matter pollution in the global South, particularly South East Asia and Brazil [Tang & Yap, 2020; Adam et al., 2021]. Therefore, shifting to more soilless and circular protein sources has an important positive interaction between mitigation of the land-related impacts discussed previously and the opportunity to greatly reduce global warming potential and atmospheric pollution associated with protein of livestock feed. Another source of GHGs from conventional crop production is associated with the main hotspots for energy and fossil fuel use, which are the operation of machinery for field operations (~63% of total energy-use) and the production of necessary synthetic fertilisers, herbicides and pesticides (~37% of total energy-use) [Hoffman et al., 2018].
Interlinked with the land-use related impacts mentioned previously, are also direct impacts to biodiversity. Disturbances of forest ecosystems (i.e., deforestation) by crop farming expansion, among other industries, is a big driver of species extinction of indigenous flora and fauna [Semper Pascual et al., 2019]. Together with restrictions enforced by several nations globally regarding the importation of crops that cause deforestation (e.g., UK Soy Manifesto https://uksoymanifesto.uk/faq/), soilless and circular alternative protein sources may present much needed opportunities towards biodiversity conservation, especially when they are implemented in regions that are considered as biodiversity hotspots e.g., Brazilian Cerrado [Lima et al., 2019; Paiva et al., 2020]. However, as previously discussed it is important that shifting to such solutions does not leave behind abandoned and degraded land. Degraded soils cannot support plant succession with a healthy organic horizon (i.e., humus) and sufficient nutrients and this can have detrimental effects on the diversity of plant species. A secondary effect of land degradation is habitat fragmentation (e.g., fragmented forest ecosystems), and so if conventional protein crops are replaced then the maintenance of patches that allow species and gene flow should be considered [Pacheco et al., 2018].
Furthermore, the use of large amounts of nitrogen and phosphorus synthetic (e.g., urea and di-ammonium phosphate) and organic (e.g., manure) fertilisers for conventional protein crop production is the primary contributor to acidification and eutrophication impacts associated with livestock feeds, both for the terrestrial and aquatic ecosystems [FAO, 2016a; Zortea, Maciel & Passuello, 2018].
Finally, agriculture is the largest contributor to water stress globally, with approximately 57% of the blue water footprint of global conventional crop production being unsustainable [Mekonnen & Hoekstra, 2020]. Oil crops (e.g., soybeans, rapeseed, sunflower) are the primary sources of protein in livestock diets requiring an average of 2400 m3 of water per ton of produce; the second highest crop class in water requirements, after pulses (e.g., beans, peas, lentils) [Mekonnen & Hoekstra, 2011].
Economic drivers
The cost of production and supply of conventional protein crops involves large financial investments associated with necessary direct inputs, including planting material (e.g., seeds), fertilisers and water for growth, and plant protection products (e.g., pesticides, herbicides). Major costs are also incurred due to labour inputs, fuel consumption for on-farm operations (e.g., tillage, spray runs), and for the land (e.g., rent) [FAO, 2016b]. Transporting large amounts of crops over long distances, often internationally and involving various means of transport (e.g., ships, planes) is another source of significant costs. In the case of soybean that comes from Brazil, which is a major global supplier, there are additional, high logistics costs due to poor infrastructure connecting the producing region to the exporting hubs, even when alternative routes are explored [Oliveira et al., 2020]. Furthermore, due to the dependency of conventional protein crop production on fossil fuel (i.e., oil, petrol), the volatility of the price of such inputs can often cause price spikes and interruptions in the supply of critical livestock protein feeds, such as soy and sunflower meals [Taghizadeh-Hesary, Rasoulinezhad & Yoshino, 2019].
Food safety and social drivers
The conventional feed production and processing system exposes protein ingredients to several biological and chemical contaminations at high rates and throughout the various phases, such as pesticides at production and fungal contaminations at storing and transportation. One particularly concerning type of such contaminations is caused by mycotoxins. These can have carcinogenic, immunosuppressive, mutagenic and teratogenic implications for animal and human health [Kabede et al., 2020]. Aflatoxin, a naturally occurring mycotoxin produced by the Aspergillus flavus fungus, is known as the most potent hepatocarcinogenic molecule in nature and can cause stunted growth, breathing difficulties, hormonal imbalances, reproductive diseases and even lead to death [Conte et al., 2020; Mahato et al., 2021]. Oilseeds including soybeans, rapeseed and sunflower seeds that are subjected to stressful conditions like prolonged heat, drought, damages by insects, and damages or moisture at transportation/storage are vulnerable to A. flavus contamination, which appears as yellow-green or gray-green mold on the seeds. If these seeds are then used as livestock feed, they pose a serious threat to animal health due to increased levels of the aflatoxin. Through livestock products such as meat, milk and eggs, humans then get exposed to aflatoxins. These contaminants are persistent and can be found even in human milk through the form of other metabolites, threatening infants’ healthy development [Alshannaq et al., 2018]. Therefore, it is important to explore solutions that allow for more resilient genotypes and shorter production and supply chains to avoid contamination risks. Furthermore, diversifying crop production with the adoption of alternative protein feeds may present further opportunities for social development as it would increase the overall size and economic value of the livestock feed production sector (currently 62% of the European cereal crops, 88% of soy, and 53% of pulses used as animal feeds) (FAOSTAT, 2021).
Local, soilless and circular alternatives as a potential solution
A wealth of research has focused on the nutritional value, digestibility, and use of alternative protein feeds for livestock. Literature suggests that alternative protein from local, soilless and circular sources can sufficiently substitute conventional feed ingredients from a nutritional perspective, although soy is still considered among the most balanced protein feeds for optimal animal growth [Gasco et al., 2019; Luciano et al., 2020]. In addition, such alternative protein sources may present solutions to address the critical sustainability issues of conventional feed production discussed above. In the following chapters, this report presents opportunities and risks associated with the incorporation of alternative protein feed ingredients sourced from:
- Genetically modified / engineered (GM/GE) protein crops and alternative protein crop growing methods (Chapter 3)
- Cellular agriculture (Chapter 4)
- Former foods, food waste, industry by-products and waste streams (Chapter 5)
- Animal by-products and insects (Chapter 6)
The classification above is in accordance with practices from recent scientific literature and was selected following consultations with experts from the FSA and the Department for Environment, Food & Rural Affairs (DEFRA). The first group represents plant-derived protein from alternative crop types (e.g., GM/GE variants, legumes) or alternative plant growing methods (e.g., hydroponics). The second group encompasses practices for mass protein extraction at a microscopic level (e.g., fungal, bacterial, micro-algal organisms). The third group represents circular agriculture streams using waste and by-products of the agricultural and other industries (e.g., brewing, bio-refinery). Finally, the fourth group includes processed animal proteins (PAPs) from by-products of livestock production or from insect farming.
Genetically modified/engineered (GM/GE) plant crops have been incorporated in livestock feed production commercially for more than 30 years. It is estimated that 70 to 90% of the GM/GE crop biomass, which includes GM soy, is consumed by livestock globally [van Eenennaam & Young, 2014]. The most common GM/GE crops that are used in livestock feeds either as protein or as energy sources are soy, maize, and potato. These feed ingredients make for up to 10%, 30%, and 5% respectively of the typical diets of different livestock species (e.g., pigs, poultry, cattle) [Flachowsky, Chesson & Aulrich, 2007]. Genetically modifying protein crops can reduce breeding times and enhance resistances to pests, weeds and plant diseases, which also translates into more cost-effective production and less expensive feed ingredients for livestock [Eriksson et al., 2018; Gocht et al., 2021].
Home-grown (i.e., locally grown) legumes such as fava beans, lupins, and peas, and cultivations of duckweed have presented an opportunity to replace conventional protein crops (e.g., soy) with protein sources that are less damaging to the environment considering significant environmental impacts such as land degradation, fossil fuel depletion, and global warming potential [Watson et al., 2017; Sherasia et al., 2018; Sońta et al., 2019]. In addition to legumes and duckweed, other promising alternative plant-based sources of high-quality proteins for feedstuffs are algae and seaweed (macroalgae), which can also provide livestock with a range of vitamins, minerals, and fatty-acids [Costa et al., 2021, Duarte, Bruhn & Krause-Jensen, 2021]. State-of-the-art hydroponics and aquaponics practices (e.g., hydroponics fodder from cereal grain) may unlock additional benefits for sustainability primarily by reducing the land footprint of protein and reliance on synthetic inputs (e.g., fertilisers) [Bartelme et al., 2018].
Environmental implications
Land degradation, land use change and land availability related impacts
Home-grown proteins could substitute sizeable amounts of imported protein that has been grown in high-risk regions like the Brazilian Cerrado, thereby relieving land-related pressures in those regions [Paiva et al., 2020]. Future climate change projections have indicated more than a twofold increase in soybean yields for areas of the North (i.e., East Canada), and so growing soybeans locally along with other local protein crops (e.g., lupins) could enhance the effectiveness of this practice to reduce land-use related impacts [Cordeiro et al., 2019]. GM/GE protein crops are more resilient to extreme climates and could help accelerate a shift of protein crop production to Northern areas [Alig & Ahearn, 2017]. Although not many studies have quantitatively investigated the direct effect of GM/GE crops on land-use change, evidence suggests that there is the possibility of unintended negative consequences due to the displacement of locally grown proteins and knock-on effects on land use [Eriksson et al., 2018]. Introducing more protein from soilless cultivations in livestock feed, such as freshwater algae and marine macroalgae (seaweed), present another opportunity in mitigating land-related impacts [Øverland, Mydland & Skrede, 2019; Koesling et al., 2021].
An issue that should be carefully considered when evaluating alternative protein sources, particularly soilless cultivations, is that of land degradation, which is largely caused by the abandonment of crop production-associated land [Winkler et al., 2021]. Steering away from protein grown in the South (e.g., Brazilian soy) without planned sustainable alternative land uses or conservation actions (e.g., reforestation) may result in vast areas of abandoned and mismanaged land. This, in combination with effects of climate change in the south such as increasing temperatures and frequencies of extreme droughts, could exert pressures on the land surface and especially the soil organic carbon [Olsson et al., 2019]. Moreover, the specific soil management practices (e.g., tillage, reduced tillage) should be considered when genetically modified/engineered and home-grown alternatives are implemented, because these can have a great impact on soil carbon sequestration and therefore soil quality [Johnson, 2018].
Greenhouse gas emissions, atmospheric pollution and fossil fuel depletion
GM/GE protein crops being resilient to plant diseases, poor soil conditions, and nutrient availability, could help reduce the production and use of chemical inputs, further mitigating such impacts. Reducing spray runs on the field and requirements for tillage (i.e., to prepare the soil for cultivation and for weed control) can help reduce fossil fuel usage by many billions of litres annually and significantly reduce associated GHGs [Brookes & Barfoot, 2020]. Hydroponics and aquaponics may present another alternative to help mitigate GHGs and fossil fuel depletion, since they are soilless cultivations and most of their energy-related impacts are associated with the use of electricity (i.e., for lighting, greenhouse and water heating), which can be generated through renewable sources. While such alternative cultivation methods could be implemented at large scales using energy sourced from fossil fuel and still reduce several environmental impacts (e.g., land-use, nutrient leaching from fertilisers), renewable energy sourcing would largely improve their pollution mitigation potential and economic viability. The use of fertilisers is also minimal with these cultivation methods and accounts for less than 2% of their abiotic depletion potential [Chen et al., 2020]. Another way to improve livestock sector carbon footprint and energy-efficiency is by obtaining protein for livestock feed from crops grown locally (i.e., home-grown protein), since using geographically shorter supply chains could help reduce emissions and energy requirements for transportation and packaging [Taelman et al., 2015].
Nitrogen and phosphorus related impacts
GM/GE crops have increased resistances to extreme weather conditions, water and nutrient scarcity among others. With these enhanced genotypes, they can supply the livestock sector with protein throughout the year with reduced synthetic fertiliser needs [Paul, Nuccio & Basu, 2018]. Further reductions can be achieved with the adoption of algae and seaweed cultivations for protein production. In addition to the fact that these alternatives do not make use of synthetic fertilisers, they absorb very large amounts of carbon, nitrogen, and phosphorus from freshwater and oceanic ecosystems that could otherwise lead to significant aquatic acidification and eutrophication impacts [Zheng et al., 2019; Gao et al., 2021]. Most of the alternatives discussed here have the potential to greatly reduce the need for such inputs and therefore, significantly mitigate relevant environmental impacts and reduce economic costs. Despite the many opportunities to mitigate nitrogen and phosphorus related impacts of livestock feed, there are some potential, relevant risks that need to be considered. Soy protein has been a very popular choice for animal production because it is very well balanced and easily digestible. Substituting soy with alternative protein feeds may result in changes in manure and urine compositions, through varied N and P amounts excreted, which in turn may lead to higher concentrations of nitrogen on fields with their application at crop production [Trabue et al., 2021].
Despite the many opportunities to mitigate nitrogen and phosphorus related impacts of livestock feed, there are some potential, relevant risks that need to be considered. Soy protein has been a very popular choice for animal production because it is very well balanced and easily digestible. Substituting soy with alternative protein feeds may result in changes in manure and urine compositions, through varied N and P amounts excreted, which in turn may lead to higher concentrations of nitrogen on fields with their application at crop production [Trabue et al., 2021].
Impacts to water quality and depletion of water resources
GM/GE crops, due to their resistances can provide abundant, healthy protein crop yields throughout the year without overburdening the available water resources with synthetic and chemical inputs [Paul, Nuccio & Basu, 2018; Dinar, Tieu & Huynh, 2019]. Reducing the use of fertilisers, pesticides, and insecticides can help improve the ecological quality of waters and prevent losses of biodiversity from the potential leaching of such chemicals in freshwater bodies and coastal ecosystems [Kumar et al., 2020]. However, recent research has also highlighted a potential opposite effect and threat of transgene sequences to be transferred to weeds, creating herbicide-tolerant superweeds. This may lead to an overall increase in the use of herbicides, which needs to be considered particularly in relation to water safety [Tsatsakis et al., 2017].
Soilless cultivation alternatives such as hydroponics and aquaponics can also help improve water use efficiency when compared to traditional crop production, particularly when exploiting wastewater resources [124 et al., 2019]. The uptake of toxic metals and other contaminants by the plant however, is a major concern that needs to be considered when wastewater is used for growing purposes [Cifuentes‐Torres et al., 2021]. Seaweed farming can improve life below water by facilitating the development of complex habitats, stimulating biodiversity, providing organic matter within and beyond the boundaries of their habitat, and converting large amounts of carbon to low carbon feed and energy [Duarte, Bruhn & Krause-Jensen, 2021].
However, it may also be the driver for negative environmental changes that need further investigation and careful consideration when planning for large-scale seaweed farming. For example, it creates competition for light and nutrients between cultivated and wild species (e.g., planktonic communities), pollution from artificial material as farming infrastructure, noise disturbances to animals due to increased vessel activity in the area and may significantly alter the geomorphology of coastal ecosystems. This is due to the absorption of kinetic energy from waves, creating microclimates that may even extend beyond the farming boundaries [Campbell et al., 2019].
The use of home-grown proteins may present an opportunity to further reduce the water footprint of livestock feed, especially if it substitutes imported protein grown in high-risk regions in terms of water scarcity (i.e., Brazilian soy from the Cerrado) [Santos & Naval, 2020].
Impacts to biodiversity
Using GM/GE crops may drive changes to agricultural biodiversity, for example reducing weed seeds diversity by up to 36% [Andow, 2003] through the mechanisms of gene and trait transfer to non-targeted, wild species, invasiveness, and weediness [Tsatsakis et al., 2017]. Furthermore, farmers tend to use more potent chemicals when herbicide and pesticide resistant GM/GE crops expand beyond domestication boundaries (i.e., weediness), leading to biodiversity losses of terrestrial and aquatic species in nearby fields and water bodies [Schütte et al., 2017]. Such effects can be disastrous to the point that close to complete mortality (96% – 100%) has been reported as the potential effect of specific chemical herbicides on North American freshwater biodiversity [Relyea, 2005].
Economic implications
Production and supply economics
GM/GE crops can help significantly reduce costs for fertilisers, water, pesticides, and herbicides, while supplying increased yields [Kumar et al., 2020]. Given their potential to be cultivated in a broader geographic range than conventional crops, they could help reduce transportation costs if produced closer to the receiving markets or to transportation hubs. Shifting to more home-grown protein alternatives like lupin, can also help reduce fuel for transportation and the associated costs, and are generally less costly to cultivate compared to traditional protein sources like soy [Lo, Kasapis & Farahnaky, 2021]. Even in scenarios where transportation fuel may rely on renewable sources (i.e., bioethanol), it is important to consider that demand may continue driving prices of biofuel feedstocks high, which include valuable conventional protein sources for the livestock sector like soy and rapeseed [Popp et al., 2016; O’Malley & Searle, 2021]. This further highlights the need to incorporate more alternative protein sources to maintain market stability and feed availability globally.
Literature is conflicted about the economic viability of seaweed farming as an alternative to sourcing protein for feed, suggesting that it is a good solution when implemented in poorer countries especially as post-harvest processing technologies become better and more affordable [Duarte, Bruhn & Krause-Jensen, 2021], but not a cost-effective industry when implemented in the Northern countries primarily due to the increased labour costs compared to the global South [van den Burg et al., 2016; Emblemsvåg et al., 2020].
The economics of alternative protein sources at large scales is a major concern for their adoption, since only a few alternative protein production and supply systems have been tested and exploited commercially to date (e.g., genetically modified/engineered protein crops). Hydroponics and aquaponics practices are mainly implemented in small scales, for example to provide single cattle farms with fodder or in urban systems for the provision of leafy vegetables, where they generate high profits [Girma & Gebremariam, 2018; Greenfeld et al., 2019]. While little research has been done to evaluate their economic performance at industry level, there may be economic benefits through integrated production systems and the co-production of protein crops and fish-meals, also relieving pressures from the demand of such ingredients both for livestock feed and human food [Goddek et al., 2015; Palm et al., 2018].
Robustness to economic uncertainties and extreme events
Among the alternatives discussed here, there are production methods that consume less energy than conventional protein crop systems and rely much more on electricity, which can be sourced from renewables, rather than fossil fuel for their needs. These include hydroponics cultivations, algae and seaweed farming, insect farming, sourcing proteins from microorganisms, and from industry waste streams and by-products. Electricity for these systems can be sourced from a variety of renewable sources, for example from biogas and solar, wind, and tidal energy, which rapidly becomes less expensive than energy from fossil fuel. Local solutions for energy sourcing can further enhance understanding and control by governmental authorities over relevant inputs and emissions associated with the agri-food sector. Therefore, considering the dependencies between fossil fuel and feed ingredient prices and the added benefits of local renewable energy, such alternative protein sources may offer resilient solutions for the future of livestock feed, particularly as policy makers continue to support the development and diversity of the renewable energy sector [Punzi, 2019].
Over the past two years, the Covid-19 pandemic has forced strict restrictions on global trading and caused a great shock to the economy of the livestock sector due to the inaccessibility of conventional feed ingredients and other necessary resources [Lioutas & Charatsari, 2021; Rzymski et al., 2021]. Local protein sources, for instance from the home-grown cultivation systems discussed here, could potentially help mitigate some of these economic impacts. Furthermore, the pandemic has raised awareness about the investment in developing automation technologies and has driven advancements in treatment practices that eliminate the risks of pathogen and disease dispersal [Henry, 2020]. As discussed above, many of the alternatives presented here could benefit greatly from such developments, which would potentially enable them to make the step to commercial, large-scale production. Aside from Covid-19 related impacts, uncertainties around global trading dynamics and future trading partners call for protein sources that are resilient to fluctuations in import / export policies and do not rely on imported resources, including imported energy [Taghizadeh-Hesary et al, 2019; Choi et al., 2021; Yao et al., 2021]. The conflict in Ukraine has already led to historical high prices for European wheat and corn, and a huge increase in the price of sunflower meals, a main protein ingredient in livestock diets [IFIP, 2022]. Furthermore, the availability of chemical fertilisers and pesticides is expected to become very limited since Ukraine and Russia are major exporters of such inputs, while their price has already almost doubled and is expected to continue to rise [Schiffling & Kanellos, 2022]. Increasing adoption of locally grown genetically modified/engineered crops, microalgae and seaweed farming, and protein from waste streams may help minimise dependency of the livestock feed production sector on such inputs, especially if these are accompanied by a shift from fossil fuel towards renewable locally sourced energy. A diversification of protein sources may help avoid cases where feed producers shift to other profitable crops (e.g., energy crops for biofuel) in times of such crises, therefore leading to a more robust livestock sector [USDA, 2022].
Social implications
Policy making in the agri-food sector often overlooks the social pillar of sustainability and considers it as a lower priority compared to environmental and economic considerations. However, as this section discusses for each alternative protein feed category, their production and use may have important social implications for animal health and welfare, food safety and public health, and social development.
Nutritional value and animal growth
GM/GE protein crops (e.g., Soybean Mon87701) can improve conventional ingredient nutrient profiles, therefore potentially improving animal growth without compromising animal and human health [Buzoianu et al., 2013; EFSA, 2020]. Studies have found that GM/GE soy can contain between 48% - 63% of crude protein, compared to the 20% - 55% average protein content that can be obtained by conventional soy crops [Edwards et al., 2000; Sauvant et al., 2004; Giraldo et al., 2019]. This shows an opportunity in that smaller quantities of GM/GE soy crops could substitute conventional soy and fulfil the relevant livestock requirements for protein. Research has also shown that the inclusion of seaweed (macro-algae) as a protein source in poultry diets can improve growth performance, laying rates and product quality [Coudert et al., 2020]. Seaweed protein contents can vary widely however, depending on the farmed species (e.g., Palmaria palmata, Porphyra sp.) between 3% - 47% [Morais et al., 2020]. Crude protein contents from homegrown legumes and duckweed can also be comparable to conventional protein feeds, ranging between 20% - 45% [Sońta et al., 2019; 2021].
Animal health and welfare
The resistant genotypes of GM/GE crops may help mitigate losses in nutritional value and more importantly potential fungal and bacterial contaminations caused by damages or decay under poor conditions of transportation and/or storage. Using local protein crops may offer another option to mitigate fungal and bacterial contaminations caused by the transportation and/or storage of feeds, particularly when these substitute ingredients that are imported from different countries and very long distances (e.g., soybeans). Transportation of contaminated feed stuffs over long distances (e.g., from China to the USA) greatly increases the risk for transmission of pathogens, including fungal toxins and viruses. Studies have shown that this has been the main pathway for transmission of animal diseases such as the African Swine Fever and Foot/Hoof and Mouth Disease across countries and even continents [Becton et al., 2022]. These diseases, although they are not contagious and harmful to humans, can cause severe impairments for animal growth thereby leading to a significantly less productive sector [Becton et al., 2022].
Social development
Home-grown protein crops could stimulate economic and social growth in local rural communities mitigating negative impacts of urbanisation [Swain & Teufel, 2017] and smallholders, local producers may acquire a more central role in the agricultural sector.
The introduction of novel technologies and practices required for the commercialisation of alternative protein sources may promote cross-sectoral knowledge sharing and collaborations, and opportunities for education as the demand for more specialised on-farm labour may increase [Marinoudi et al., 2019]. On-farm work safety could be improved significantly through production methods that minimise the use of hazardous agrochemicals (e.g., pesticides, herbicides, chemical fertilisers) and that rely on automated technologies [Elahi et al., 2019]. Through increased efficiency, reduced on-farm risks, and reduced demand for heavy physical labour, alternative protein production chains may contribute to an overall improvement of labourer welfare and gender representation in the livestock sector.
Furthermore, the alternative protein sources presented in this section have the potential to mitigate greatly negative impacts on terrestrial and aquatic ecosystems and biodiversity, and therefore preserve ecosystem services improving firstly human wellbeing and quality of life, but also global agricultural growth [Rukundo et al., 2018]. This is particularly important when considering the extent of damages to some of the planet’s most valuable and pressured ecosystems such as the Brazilian Cerrado and Amazon’s and Borneo’s rainforests, whose services are appreciated globally [Flach et al., 2021]. As previously discussed, shifting to local protein crops, growing genetically modified/engineered crops in the global North, or adopting more of the landless cultivation methods presented here, may help reduce pressures and contribute towards the conservation of such important ecosystems [Weindl et al., 2020].
Consumer perception and acceptance
Consumer perception has always been a big concern and a barrier to the adoption of alternative proteins both for human consumption and for livestock feed. Although meat consumers and livestock farmers seem to be positive about alternatives such as GM/GE crops, insects, algae, and lab-grown feeds used in livestock production there is still much to be explored regarding the tipping point in acceptance and the specific factors that affect it [Verbeke et al., 2015; Onwezen et al., 2019]. Providing sufficient and credible information with the products at the point of sale is critical to build trust with the consumers and facilitate their habituation and acceptance towards alternative feeds [Altmann et al., 2022; Khaemba et al., 2022]. Issues of mislabelling need to be controlled and avoided particularly as long as GM/GE protein ingredients are perceived as having safety issues by some consumers [Montgomery et al., 2020]. Feed and food fraud threaten customer trust and acceptance, but also food security since they often exclude vital information about potential sources of fungal, bacterial, or chemical contamination. Other studies however have shown that overly exposing the public to risk assessment protocols for relevant modern biotechnologies and procedures (e.g, transgenesis) may contribute to feelings of distrust and fear regarding the safe use of GM/GE feeds for livestock [Giraldo et al., 2019].
Food safety
The use of GM/GE protein crops as livestock feed can have unintended negative implications for human health. The safety of most transgenic protein feeds has been evaluated in the context of “direct use as human foods” because they can also be consumed by humans (e.g., soybeans, canola). However, more research is needed to understand, monitor and regulate the risks for toxicity and allergenicity of GM/GE livestock feeds to humans through indirect exposure by consumption of livestock products [Giraldo et al., 2019]. An unintended negative impact is horizontal transfers of genes from GM/GE protein foods, the presence of which has been reported in the digestive tract of humans; this does not exclude the possibility for a transfer to humans through livestock meat products. However, the quantities recorded have been relatively small (i.e., maximum ~4% of transgenic DNA) and studies suggest that the low pH in animals’ stomach can degrade most of it before large quantities reach humans [Netherwood et al., 2004; Dona & Arvanitoyannis, 2009; Korwin-Kossakowska et al., 2020]. Another potential threat to human health may arise indirectly through the overuse of glyphosate (herbicide) that many farmers do to combat weediness of herbicide resistant GM crops; accumulated glyphosate in plant tissues and root system promotes the growth and mycotoxin production of the fungi Fusarium which can reach humans through the pathways described above [Diaz-Llano & Smith, 2006].
Aside from the adoption of protein sources that are more resilient to biological contaminants, minimising mycotoxin outbreaks starts with good management practices such as thorough grain/seed cleaning, removal of damaged seeds and debris, and sanitation of handling and storage equipment [Ráduly et al., 2021]. However, considering that pressures of climate change and unavoidable transportation/storage issues (e.g., Ukraine-Russia conflict) can greatly affect the growth and distribution of contaminants and the risks they pose to feed and food safety, it is critical that we employ multiple controlling mechanisms including alternative protein sources that are grown more locally [Magnoli et al., 2019].
Finally, recent research has shown that there is no evidence to suggest that GM/GE protein crops expose humans to novel allergens or that they are more allergenic than the conventional counterparts [Dunn et al., 2017]. On the contrary, some studies propose that GM/GE feeds and foods may even reduce the expression of proteins that lead to allergic reactions, therefore being more suitable for human consumption from this perspective [Dubois et al., 2015]. Overall, more research is required to precisely evaluate the risk for severe allergic reactions caused by animal feeds; for example, how potent can protein meals from fava beans be as an allergen for individuals with G6PD deficiency? The inclusion of seaweed in feeds may also lead to allergic reactions, similar to those exhibited due to intolerances in seaweed-extract food additives (e.g., carrageenan from red seaweed) [Santo et al., 2020].
Regulatory implications
While the popularity of GM/GE protein feed ingredients has greatly increased over the past two decades, incorporating them at commercial scales in livestock feeds requires thorough safety assessment and labelling protocols. The US and Canadian regulatory authorities perform comparisons with conventional counterparts through scientific experimentation to evaluate the safety of GM/GE products, while legislation in EU focuses more on controlling and certifying the modification process. In all cases however, it is important that the regulatory system knows the actual genes that are being transferred to the feed crop and understands potential changes in its functionality (e.g., production of novel protein / enzyme with potential allergenic action) [Giraldo et al., 2019]. Besides GM/GE protein feeds, labelling and traceability protocols are important also for the use of homegrown legumes and seaweed, especially when considering potential risk of allergies and intolerances.
Similar to algae and seaweed, micro-algae are rich in fatty acids and carotenoids important to livestock from aquatic species (e.g., salmon, shrimp) to poultry and pigs and to humans who consume it mainly in the form of food supplements [Jones et al., 2020]. Fungal proteins (e.g., yeast protein) have long been used both in feeds and human foods and current research focuses on ways to optimise their production. One particularly interesting field of research looks into the potential for animal and agricultural food waste (e.g., fish, fruits) to be used as substrates for fungal protein production, therefore upgrading waste into high-value products while supplementing the livestock sector with important protein resources [Tropea et al., 2022]. Bacterial protein is another well-known alternative solution for livestock feed, which can benefit from further developments in upgrading waste or by-product substrates particularly in reducing production costs to enable it to compete with less expensive conventional feeds [Puyol et al., 2017; Ritala et al., 2017].
Cellular protein is typically produced through the ability of microorganisms to convert organic and inorganic carbon substrates into valuable protein and other nutrients, or through the use of GM/GE technologies (i.e., bioengineering) to extract such macromolecules from fermented cell cultures (e.g,. Quorn, cultivated soy-meat).
Environmental implications
Land degradation, land use change and land availability related impacts
Production of protein feeds from cellular sources is not dependent on arable land and therefore presents an effective solution to improving overall resource efficiency of the livestock sector and mitigating land-use related impacts [Nyyssölä et al., 2022]. As cellular agriculture becomes increasingly popular in the global North, it can significantly help relieve land-use pressures in high-risk forested areas of the South [Stephens & Ellis, 2020]. Cellular protein can be produced in facilities located in urban areas (e.g., labs, vertical farms), consequently freeing up agricultural land for food crops and livestock.
Greenhouse gas emissions, atmospheric pollution and fossil fuel depletion
Cellular agriculture relies less on fossil fuel than conventional protein crop production and currently operates on a mixture of fuels, consisting mainly of natural gas, coal and electricity from renewable sources; a study using US large scale cell production as a case in point indicates a fuel blend of 43% : 33% : 16% respectively [Mattick, 2018]. As a result, substituting protein crop feeds with cellular protein can help reduce fossil fuel depletion and associated GHG emissions to atmosphere. The potential for mitigation of such impacts can be increased by enabling additional usage of renewable energy sources for cellular agriculture shifts, especially since its main operations and energy requirements are for heating and artificial lighting and their automated regulation [Mattick, 2018]. Bacterial and fungal protein (e.g., methanotrophs and yeasts respectively) can reduce GHG emissions and improve resource efficiency of livestock feeds further through the utilisation of industrial wastes and residues as feedstocks, for example CH4 and CO2 emissions from oil drilling [Nyyssölä et al., 2022]. Finally, since cellular protein production can take place in urban areas and close to feed processing / manufacturing facilities and distribution hubs, it can reduce GHG atmospheric emissions and fossil fuel use associated with transportation requirements.
Nitrogen and phosphorus related impacts
Unlike conventional crops, cellular agriculture does not require synthetic fertilisers to provide the necessary nutrients for protein production. Instead, nitrogen fixating microorganisms can either recycle the reactive nitrogen available on soils and organic / inorganic substrates, or fixate atmospheric nitrogen [Helliwell & Burton, 2021; Nyyssölä et al., 2022]. These processes help reduce surpluses of nitrogen and phosphorus that could potentially lead to acidification and eutrophication of terrestrial and aquatic ecosystems.
Impacts to water quality and depletion of water resources
The water footprint of protein production from microorganisms can vary greatly depending on the type of substrate used and location of production. In cases where cellular agriculture uses plant-derived feedstuffs (e.g., sugar), total consumption of water (including requirements for climate regulation, heating and cooling of the facilities) is comparable to that of conventional crop production [Behm et al., 2022]. On the other hand, cellular agriculture generates significantly less wastewater than conventional protein crops and therefore it does not threaten water quality. Moreover, it can utilise a range of water streams such as fresh, marine and recycle wastewater, further reducing pressures on available water resources [Nyyssölä et al., 2022]. However, to best evaluate the effectiveness of this strategy to improve water quality, alternative pathways for food waste recycling should also be considered (Fig. 3) [Puyol et al., 2017].
Figure 3: Different biological technologies and pathways for wastewater treatment and recovery. Source: Puyol et al. (2017).
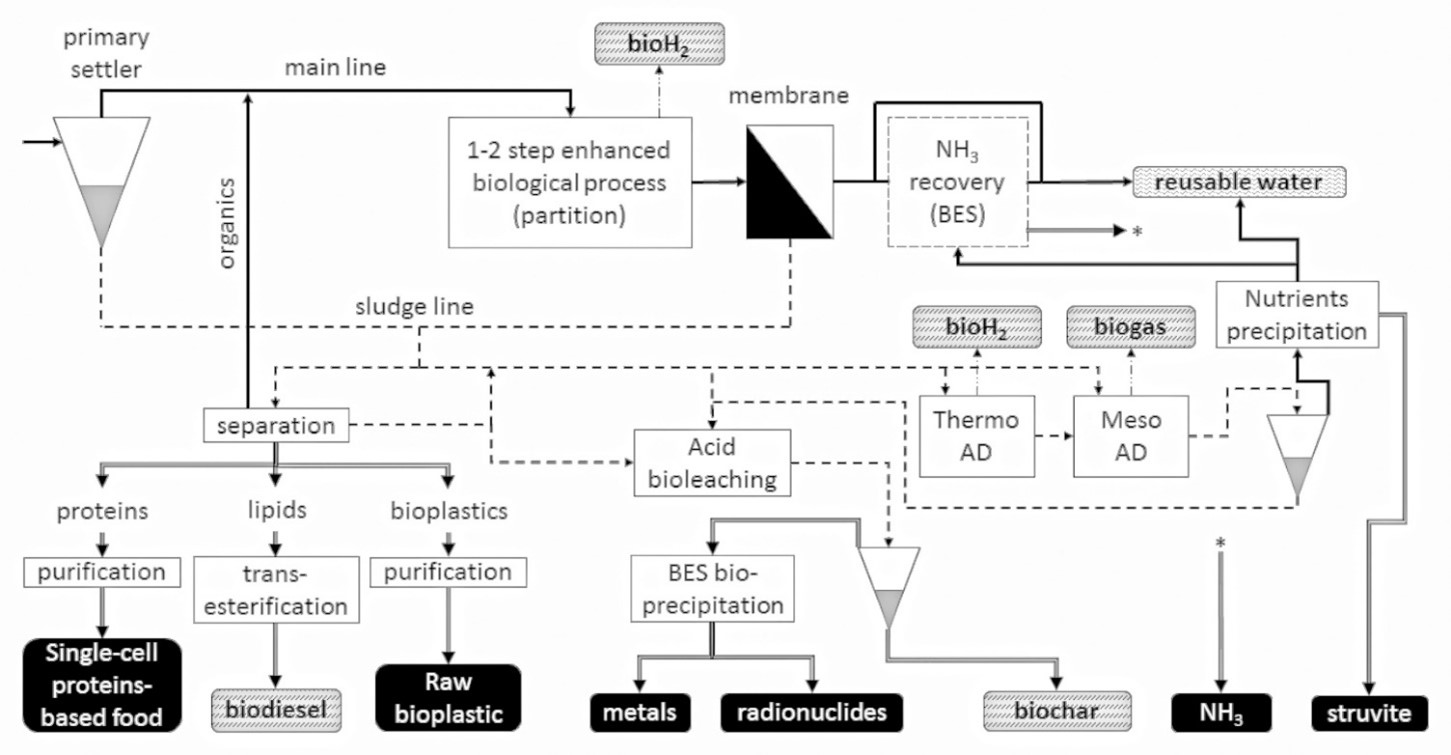
Impacts to biodiversity
Cellular agriculture contributes to the conservation of biodiversity and preservation of biodiversity hot-spot areas of the planet by reducing land requirements for protein feed production and therefore mitigating associated impacts of deforestation and habitat fragmentation / loss. However, an important consideration here is that a potential abrupt abandonment of agricultural landscapes due to a shift towards cellular agriculture may lead to a loss of grassland biodiversity and habitat fragmentation if land is mismanaged [Helliwell & Burton, 2021; Moritz et al., 2022].
Economic implications
Production and supply economics and resilience to extreme events
While more research is required to quantify the costs associated with the production of protein feeds from cellular agriculture particularly under varied production scenarios (e.g., different substrates), studies have looked into the economic of cultured meat production systems and found that they can be comparable to conventional livestock and crop production systems [Bapat et al., 2021; Eibl et al., 2021]. Advancements in biotechnology can help overcome challenges regarding the large capital investments required for production (e.g., purchasing of production-related equipment), for treatment of waste substrates to enhance biosecurity, and for sourcing of required specialised labour [Odegard & Sinke, 2021]. Such developments will make cellular protein more competitive in the feed market and help address current market uncertainties related to the willingness of feed manufacturers and livestock producers to shift away from conventional protein sources [Saavoss, 2019].
The relatively low fossil fuel requirements of cellular agriculture and ability of microorganisms to convert a range of waste streams to valuable products enables novel circular economy pathways that enhance resilience of the livestock feed production sector against fluctuations in fuel prices and prices of relevant agricultural commodities (e.g., plant-derived feedstuffs). If more and less expensive renewable energy becomes available for cellular agriculture (e.g., electricity from solar power), then its economic performance at large scales can be further improved [Eibl et al., 2021; Helliwell & Burton, 2021; Nyyssölä et al., 2022].
Considering the uncertainties and instabilities in global fuel prices and trading policies, the ability of cellular agriculture to take place in urban areas and close to distribution hubs may greatly reduce costs associated with transportation and increase feed security and availability in local livestock production systems. Finally, cellular agriculture allows for better control of the levels and quality of production, therefore providing higher consistency of representation in the market and financial security [Gasteratos, 2019].
Social implications
Nutritional value and animal growth
From a nutritional perspective, protein from cellular agriculture sources can sufficiently compare to protein levels from conventional sources. Specifically, crude protein contents from fungi (e.g., Saccharomyces cerevisiae), bacteria (e.g., Arthrospira plantensis) and micro-algae (e.g., Chlorella vulgaris) can range from 33% to 47%, 51% to 81%, and 7% to 59% respectively [Pignolet et al., 2013; Glencross et al., 2020]. Research has shown that the amino acid composition and nutritional profile of cellular protein meals that have been tested in livestock and fish feed formulations does not lead to significant differences in animal body compositions and growth [Hardy et al., 2018; Jones et al., 2020].
Animal health and welfare
Several studies have shown that cellular agriculture can have positive implications on animal health and welfare. The control that cellular agriculture offers over the quality of produced feed (i.e., GM/GE techniques that allow for specific proteins to be produced) can provide balanced nutrients for optimal animal growth and meat quality (e.g., reduced unnecessary fats) [Mattick 2018]. Moreover, cellular protein production typically takes place under hygienic conditions in controlled environments (e.g., lab-like facilities), therefore reducing the risk of potential biological contaminations (e.g., mycotoxins) that can threaten animal health. However, safety risks that include biological and chemical contamination may arise if the substrates (e.g., crop by-products) and facilities (e.g., fermentation tanks) are contaminated during production, or if the produce is contaminated at handling and post-production processing [Teng et al., 2021]. These risks become particularly relevant in the absence of technologies required for the monitoring and detection of undesirable microbial communities, toxins and chemical pollutants (e.g., nanoplastics), especially if waste substrates are used for production.
Finally, acceptability by livestock of such novel feeds can present an issue for animal welfare. For example, characteristics of novel feeds like palatability or texture compared to conventional protein feeds may cause livestock to consume less quantities and therefore also risk good animal health and growth [Mainardes & DeVries, 2016]. However, small adaptations in management practices (e.g., social feeding strategies, gradual adaptation) can help greatly increase the willingness of livestock to consume alternative feed ingredients [Mainardes & DeVries, 2016].
Social development
Several opportunities for social development may arise with the development of cellular agriculture markets. Novel protein production systems could be located adjacent to sources of waste and industrial by-products, therefore establishing efficient waste-to-product chains and greatly improving waste disposal [Williams, 2021]. Cellular protein production facilities that are located in urban areas can help build more resilient and resource-efficient localised livestock systems that do not rely on transportation of ingredients over long distances and contribute to the development of local economies. The requirements cellular agriculture has for specialised labour and renewable energy sources can further encourage social and economic growth of less developed rural communities.
Consumer perception and acceptance
Consumer acceptance is not considered a serious threat to the marketability and economic viability of cellular protein feeds, since cellular agriculture has become an established practice in the food industry over the past two decades. Consumers have gained significant exposure to foods arising from cellular agriculture processes, especially used in vegetarian and vegan diets, which should enable the smooth incorporation of such alternatives in livestock feeds [Teng et al., 2021; Zollman Thomas & Bryant, 2021].
Potential risks regarding the willingness of consumers to try livestock products reared with cellular protein may arise when waste substrates or GM/GE – bioengineering techniques are used that are not well-understood or trusted by sections of the public. Further educating consumers on the overall environmental, economic and social benefits of this alternative, on wrong perceptions of related food safety issues, and adhering to transparent labelling protocols can help enhance consumer acceptance and adoption rates.
Food Safety
As also discussed for animal health and welfare above, a major concern for food safety arises when cellular protein production uses waste substrates that have not been thoroughly processed. Such practices introduce the risk for several biological (incl. viral, bacterial, or fungal microorganisms) and chemical contaminants (incl. microplastics, heavy metals, synthetic molecules) to enter the human body through bioaccumulation. While current research is constantly uncovering new information, there is still much uncertainty around the precise mechanisms through which such contaminants are enabled and the specific problems they may cause to humans.
Another concern for food safety relates to that synthetic protein may lead to the introduction of allergens in the human food chain similar to conventional protein sources, therefore leading to potential allergic reactions and intolerances through the consumption of livestock products [Kuhad et al., 1997].
Regulatory implications
A strict regulatory system must be in place to address safety related issues particularly when waste substrates and GM/GE techniques are used for cellular protein production. Early and precise detection of biological and chemical contaminants, as well as monitoring of good hygiene practices are critical to ensuring feed and food safety. Anticipatory monitoring strategies, such as randomised sampling and thorough inspections of production chains, as well as strict labelling policies should be developed to prevent feed and food fraud incidents, which may increase as quality and affordability of cellular protein improve and the market expands.
Supplying livestock directly with human inedible protein from food waste has been another major topic of research as a potential solution to livestock feed sustainability without compromising food availability, since approximately 30% of global arable land produces food that is subsequently not eaten [FAO, 2013; Tonini et al., 2018].
The assessment distinguishes between food waste and former foods. The former, refers to edible material that was discarded either by choice or due to events like spillage and spoilage during production and processing, while the latter refers to foods or food ingredients that did not reach retailers and consumers (e.g., due to not meeting quality criteria). It is important to consider that food waste may contain some inedible material mainly due to packaging residues [Tavill, 2020].
Many studies have investigated the nutrient profiles of popular food wastes globally, the impact of waste processing methods on the effectiveness of food wastes as feed, and the potential implications on livestock performance across several species [Dou, Toth & Westendorf et al., 2018; Luciano et al., 2020]. Evidence suggests that food waste from both hospitality and households have potential to substitute protein feedstuffs, while former foods from bakery and confectionery can be used as a source of protein in livestock diets [Pinotti et al., 2021; Rajeh et al., 2021]. Many large European and UK retailers, including Tesco, Arla Foods, and Coca Cola, have already started investing in their waste sorting procedures to safely use surplus food as animal feed [WRAP, 2016]. Such actions however have been delayed by significant food security threats, including the Covid-19 pandemic, that forced retailers, restaurants, hotels and cafeterias to operate at very low capacity, therefore generating limited amounts of food waste.
Crop residues, agro-industrial by-products, and agroforestry plants (e.g., tea, cassava, mulberry, mesquite) also present opportunities to source proteins for use in livestock feed. These sources refer to by-products that are inedible for humans, such as plant foliage and by-products (e.g., leaf protein concentrates) derived from agroforestry and post-harvest crop processing and that can be used as sources of protein, energy and fibre for livestock [Tallentire et al., 2018; Li et al., 2019; Salami et al., 2019]. Distillers’ dried grains with solubles (DDGS), a by-product of the biofuel industry, has been among the most popular examples used to substitute soya and maize in diet formulations for livestock and fish [Makkar, 2018]. Due to the potential use of such by-products as livestock feed, synergies and integrations between biorefineries and livestock systems have been explored to address issues of land availability and redistribute the environmental pressures associated with the two industries [de Souza, Junqueira & Cavalett, 2021].
Agri-food waste-streams present many opportunities to sourcing protein for livestock feed, while at the same time addressing a range of environmental pollution issues. Using novel biotechnologies, nitrogen recovery rates of close to 100% have been reported from waste and wastewater through to microbial protein for cellular protein, revealing the potential for biobased circular economies in agriculture [Puyol et al., 2017]. Recent research has also explored the potential of waste from livestock (e.g., liquid slurries) and biorefineries (e.g., anaerobic digestate) to help grow sustainable duckweed, minimising water use for its cultivation and land otherwise used for the disposal of the excess slurry [Stadtlander et al., 2019; Sońta et al., 2020].
Environmental implications
Land degradation, land use change and land availability related impacts
Generating protein from former foods, food waste, and industry by-products can help mitigate global livestock sector land-use footprint, as it alleviates pressures from land that would otherwise be used for feed-crop production. Funnelling food and industry wastes into livestock feeds can significantly reduce requirements for landfills and relieve overall pressures on waste disposal systems [Tonini et al., 2018]. Land occupied by plant, cereal and fruit production in particular, can be used to accommodate grazing needs by ruminants, in addition to reducing crop land requirements [Schader et al., 2015; Salami et al., 2019].
Greenhouse gas emissions, atmospheric pollution and fossil fuel depletion
Treating waste streams as valuable by-products helps significantly reduce the overall environmental footprint of the industries that generate them – i.e., bakery, hotels and restaurants, crop production, brewery, biofuel, etc. This is because the allocation of emissions and pollutants generated through production, including GHG atmospheric emissions and impacts to resource availability, would change from an “only the primary product is responsible” allocation process, to “emissions are allocated to the primary product and valuable co-products”. Incorporating such soilless protein sources in livestock feed frees up land and resources for conventional protein crops like soybean, which can be used directly for human consumption or by other industries (e.g., as feedstock for biofuel), overall reducing the environmental footprint of livestock feed production. Studies have shown that using food waste as feed is the best performing disposal strategy from an environmental perspective, when compared to the use of food waste as feedstock for anaerobic digestion (i.e., bioenergy) and composting (i.e., organic fertiliser) (Fig.4) [Vandermeersch et al., 2014; Salemdeeb et al., 2017; Dou, Toth & Westendorf et al., 2018].
With these alternatives, it is important that any required pre-treatment methods for the different potential uses for such waste-streams are considered as their environmental impacts and resource requirements may vary significantly (Fig. 5).
Figure 4: Environmental burdens and benefits of different uses for food waste – as dry (grey) and wet feed (brown) for livestock production, through anaerobic digestion (blue), and composting (green). The following environmental impact categories are considered: GWP = Global Warming Potential, ODP = Ozone Depletion, HT-C = Carcinogen Emissions, HT-NC = Non-carcinogenic Toxin Emissions, IR = Ionising Radiation, POF = Photochemical Oxidant Formation, FEP = Freshwater Eutrophication Potential, MEP = Marine Eutrophication Potential, ET = Ecotoxicity, ADP-F = Fossil Fuel Depletion, ADP-E = Abiotic Resource Depletion, AP = Acidification Potential, TEP = Terrestrial Eutrophication Potential, PM = Particulate Matter Emissions. Source: Salemdeeb et al. (2017)
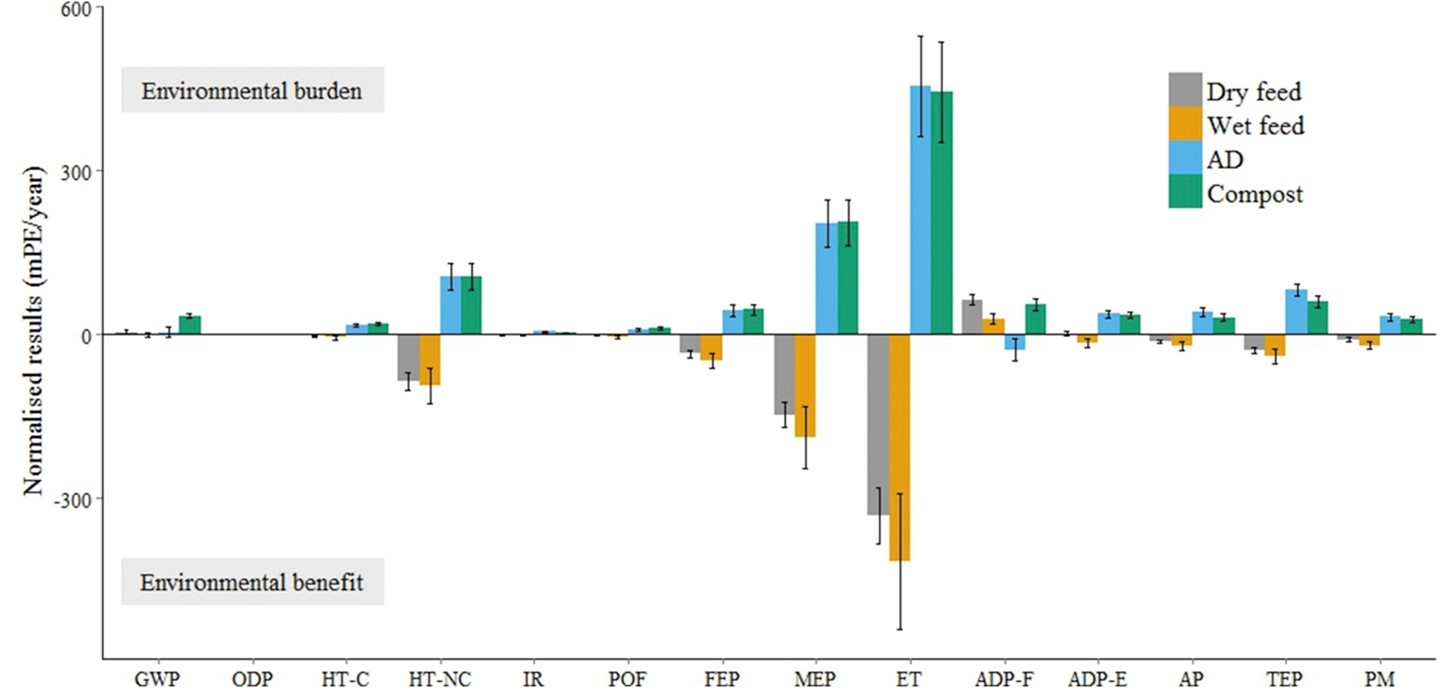
Figure 5: Fossil fuel requirements for the production of dry and wet feeds from food waste. The figure highlights the importance to consider relevant treatment and processing requirements associated with different disposal strategies for food waste, even when comparing different animal feeding strategies. Source: Salemdeeb et al. (2017).
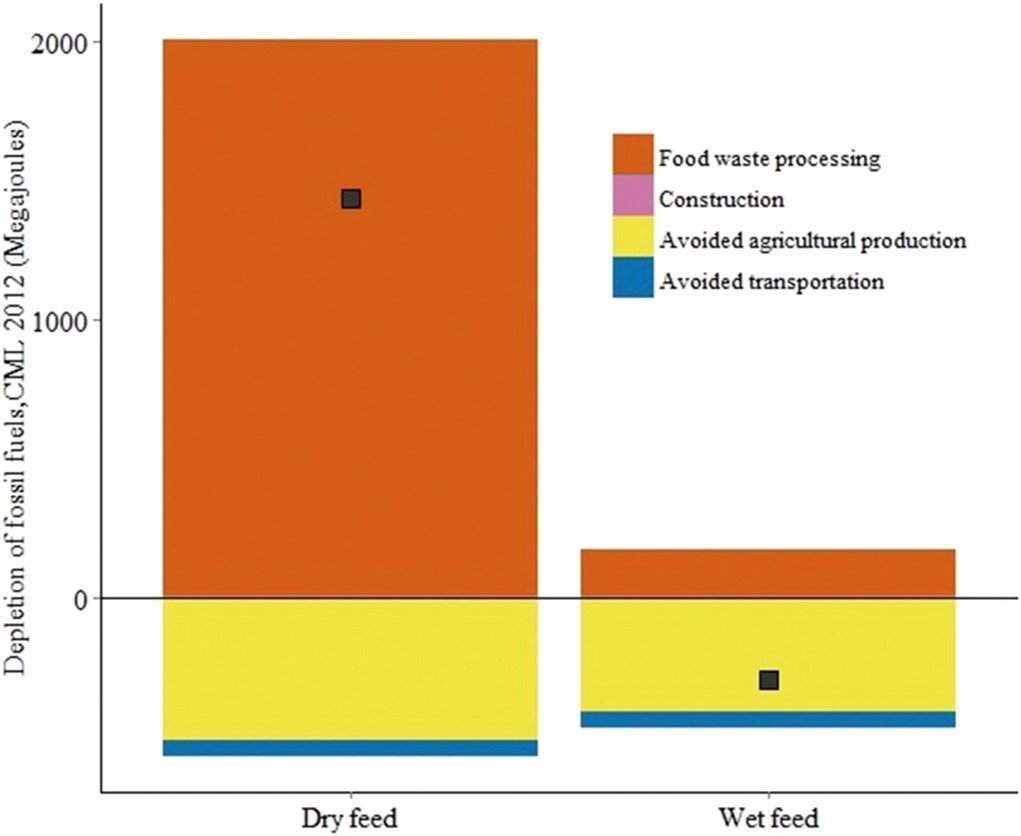
Nitrogen and phosphorus related impacts
Utilising former foods and food waste to source protein creates opportunities towards reducing acidification and eutrophication impacts, even when compared to other potential exploitation avenues (e.g., anaerobic digestion for energy production, composting as organic fertiliser) (Fig. 2) [Salemdeeb et al., 2017]. Production of feed from food waste is not dependent on synthetic fertilisers and other chemical inputs, therefore reducing pressures on fresh and marine water bodies from nutrient and chemical leaching. Furthermore, it enables a recycling path for nutrients that would otherwise be deposited to soils and aquatic ecosystems through waste disposal.
Impacts to water quality and depletion of water resources
Large improvements in water efficiency of livestock feeds can be achieved through sourcing of protein from food waste and industrial waste streams, while at the same time and through the same process removing unwanted contaminants from fresh and marine water bodies [Dou, Toth & Westendorf et al., 2018]. Substituting conventional feeds with biofuel, agroforestry, and plant by-products may help reduce the water footprint of these production chains overall, provided that increased demand of such co-products does not drive overall increases in production [Mekonnen & Hoekstra, 2020].
Impacts to biodiversity
The biodiversity impacts related to the disposal of food waste are far smaller than the ones related to the production of food that is eventually wasted [FAO, 2013]. Therefore, using food waste as feed yields its greater benefits for biodiversity conservation indirectly, sharing the environmental burden associated with food production as a valuable co-product (i.e., re-allocation of environmental impacts). Direct, positive impacts on biodiversity result from the fact that converting food waste to feed does not require any additional land or synthetic and chemical inputs compared to conventional agriculture, which helps preserve good habitat quality and reduces habitat fragmentation.
Economic implications
Production and supply economics and resilience to extreme events
Circular agriculture streams for protein sourcing, such as feed derived from food waste and former foods, may also help the livestock sector avoid large costs associated with conventional protein crop production. A critical condition for these alternatives to be viable and cost-effective is the proper treatment of food waste prior to their use as feed, to reduce the risks of pathogen and disease outbreaks that could lead to severe economic consequences for the livestock sector [Dou, Toth & Westendorf et al., 2018]. Ensuring high hygiene standards through timely collection of the waste, thorough thermal treatment, appropriate transportation and handling practices incurs costs that need to be accounted for when evaluating the feasibility of such feeding strategies [Pinotti et al, 2020; Rajeh et al., 2021]. When comparing potential uses of food waste, specifically processing it for the production of energy (i.e., anaerobic digestion) or processing it for the production of animal feed (i.e., hygienic / thermal processing), the latter incurs twice the cost [Martinez-Sanchez et al., 2016]. However, the financial benefits the livestock sector gains by reducing animal feed production this way, generally outweigh the costs and make it a potentially viable alternative [Martinez-Sanchez et al., 2016; Pinotti et al., 2021]. Further advancements in biotechnology for waste treatment may increase the cost-effectiveness of circular agriculture alternatives (e.g., protein from food waste, insects reared on waste) and cellular agriculture protein streams (e.g., bacterial protein produced from waste substrates), thereby enabling easier implementation at large scales [Ritala et al., 2017; Jones et al., 2020].
Social implications
Nutritional value and animal growth
Food waste can be a good source of amino acids, minerals, fatty acids, and vitamins essential for animal growth [Rajeh et al., 2021]. However there is no clear evidence that it can significantly improve animal performance. Literature suggests that food waste does not affect meat quality, while some studies propose it may even improve it [Dou et al., 2018]. Table 1 below as adapted from Rajeh et al. (2021), summarises the potential for food waste streams to provide valuable nutrients for animal growth when incorporated in livestock feeds.
Table 1: Nutritional value of various sources of food waste used as livestock feeds and tested on various livestock species. Adapted from Rajeh et al. (2021).
Food waste | Tested livestock | Dry matter % | Crude protein % | Crude fibre % | Optimal inclusion level (% of total feed) | Feed conversion ratio (kg intake/kg weight gain) |
---|---|---|---|---|---|---|
Retail food waste | Fish | 97.9 | 19.6 | - | 20 | 1.5 |
Restaurant food waste | Fish | 91.1-97.9 | 18.9 - 31.1 | 0.8 - 15.3 | 25 - 75 | 1.1 - 1.3 |
Hotel food waste | Fish | 93.2-97.9 | 19.6 - 31.1 | 5.7 | 20 - 53 | 1.5 - 2.6 |
Pasta waste | Fish | 89.8-91.5 | 12.7 - 14.3 | 0.3 - 2.0 | 30 - 75 | 0.9 - 2.5 |
Date waste | Fish | 86.7-92.9 | 2.4 - 2.6 | 1.8 - 2.1 | 30 | 1.2 - 1.8 |
Biscuit waste | Chickens | 89.3-92.0 | 5.3 - 12.6 | 1.1 - 2.6 | 24 - 50 | 1.9 - 2.5 |
Restaurant food waste | Chickens | 82.1-93.7 | 15.8 - 16.0 | 2.0 - 8.9 | 10 - 20 | 3.3 - 4.6 |
Retail food waste | Chickens | 82.1 | 15.8 | 2.0 | 20 | 4.6 |
Kitchen (household) food waste | Chickens | 87.6 | 15.8 | 10.8 | 5 | 3.9 |
Fruit waste | Chickens | - | 6.9 | - | 12.6 | 2.0 |
Date waste | Chickens | 95.2 | 8.1 | 9.1 | 10 | 2.3 |
Noodle waste | Chickens | 94.7 | 8.8 | 1.5 | 50 | 1.9 |
Retail food waste | Cows | 94.5 | 20.0 | - | 25 | - |
Kitchen (household) food waste | Cows | 85.3 | 20.1 | 9.7 | 50 | 7.3 |
Cafeteria food waste | Cows | 46.1 | 29.4 | - | 50 | - |
Vegetable waste | Goats | 28.7 | 8.3 | - | 82 | 33.5 |
Restaurant food waste | Pigs | 19.1-96.1 | 14.4 - 25.0 | 2.3 14.5 | 20 - 50 | 3.0 - 5.6 |
Fruit waste | Pigs | 93.6 | 19.5 | 6.2 | 4.0 | 3.2 |
Kitchen (household) food waste | Pigs | 23.1 | 14.1 - 19.1 | 4.2 - 4.4 | - | 3.0 - 3.1 |
Bakery waste | Pigs | - | 9.5 | 2.3 | 24 | 3.2 |
Biscuit waste | Pigs | 84.5 | 8.7 | 0.3 | - | 3.2 |
Cafeteria food waste | Pigs | 22.4-89.7 | 17.8-21.4 | - | 20 - 50 | 3.4 - 4.3 |
Retail food waste | Pigs | 89.7 | 17.8 | - | 20 | 4.3 |
Restaurant food waste | Sheep | 89.5 | 14.7 | - | 15 | 6.9 |
Kitchen (household) food waste | Sheep | 89.5 | 14.7 | - | 15 | 6.9 |
Biscuit waste | Sheep | 96.9 | 9.7 | 2.1 | 25 | 5.0 |
Animal health and welfare
Food waste and former foods need to be very carefully treated with thermal processing (e.g., hydrothermal treatment at 110 °C for an hour) before they are fed to animals, because they can contain viruses and bacteria that may cause serious animal diseases like swine fever and foot-and-mouth disease. Even when food waste, former foods and industry waste streams are safe from a microbiological perspective (e.g., from microbial and fungi contaminants) they may contain high levels of chemical residues from packaging (e.g., plastic) and processing (e.g., heavy metals, pesticides) causing harm to farmed animals [Pinotti et al., 2019]. Some former foods and food waste may contain high concentrations of secondary metabolites or toxins, for example chocolate residues can be high in theobromine. For this reason, their levels of inclusion in livestock feeds need to be carefully considered.
While literature suggests that packaging remnants in former foods and food waste are usually negligible (i.e., less than 0.1g per 100g), it is important to consider that different types of materials may exhibit very different resistances. For example, polyethylene and polypropylene which are two common packaging plastics, have different melting points at ~120°C and ~240°C respectively.
Social development
Upcycling of food wastes and waste streams through livestock feeds creates several opportunities for social development boosting growth of local communities, driving innovation in biotechnologies and creating jobs for specialised labour [European Commission, 2015]. Adopting circular alternatives helps improve ecosystem services, enhance quality of ecosystems, conserve habitats for wildlife, and reduce pressures from agricultural intensification for conventional crop production, which improve overall quality of life particularly for rural communities [Popescu, 2019; Zhu et al., 2019; Jagtap et al., 2021].
Consumer perception and acceptance
Evidence suggests that consumers of livestock products are currently conflicted with regards to the suitability and safety as livestock feeds of food wastes, former foods and industry waste streams, and insects reared on such waste streams. On one hand, studies have shown that consumers understand the benefits and appreciate the feeling of “doing good” by generating valuable nutrients while reducing waste and improving the environmental and economic performance of global livestock systems (i.e., “Eco-feed” concept) [Saito et al., 2009; Borrello et al., 2017; Bhatt et al., 2018]. On the other hand, the possibility that food waste can be contaminated by pathogens that caused recent outbreaks of diseases like Covid-19 and African Swine Fever, raises issues of distrust regarding hygiene standards in livestock facilities, and discouragement among livestock producers and consumers from supporting adoption of this circular alternative [Mens et al., 2021; Jayathilake et al., 2022].
Food safety
As also discussed in previous chapters, microplastics found in food wastes can reach humans bodies posing serious threats such as genotoxicity, inflammations and cell apoptosis. Besides the pathway for human exposure to microplastics through ingestion of contaminated livestock products, studies have identified another indirect and even more potent route. Specifically, research has shown that digested microplastic, i.e., microplastic that has been fed to livestock through food waste feeds and then excreted after digestion, has altered properties that increase its adsorption capacity. This means that once released in wastewaters or waste substrates through manure disposal it can be available as accidental feed for a wider group of organisms (e.g., fish, insects) and eventually reach humans through several bioaccumulation pathways [Krasucka et al., 2022].
An important risk for food security arises with the potential for several allergens to reach human foods through the use of food waste as animal protein feeds. It is important to consider that waste from restaurants, households, retailers, cafeterias, and bakery and confectionery frequently contain the majority of the 14 major allergens – gluten, dairy, fish and egg proteins, and traces of nuts, groundnuts and peanuts [ACAF, 2009; Testa et al., 2017; Bingemann et al., 2019]. Aside from allergens, food waste can contain various additives and processed ingredients, such as artificial sweeteners (e.g., aspartame), flavour enhancers (e.g., monosodium glutamate), artificial colour additives and others, which can reach humans through bioaccumulation in livestock tissues. These chemical substances have been associated with harmful effects on humans including respiratory and skin allergic reactions and even stomach and intestinal cancer [Gultekin et al., 2020; Rinninella et al., 2020].
Regulatory implications
The safe adoption of food wastes and waste streams as animal feeds presents a great challenge for regulatory systems of the global livestock sector. Similar to the case of cellular agriculture, it is critical that safety assessment protocols use state-of-the-art biotechnologies to detect biological and chemical contaminants in food waste early and with precision. There is a need for sensitive detection and treatment methods to ensure safe implementation of such alternatives, as studies have reported concentrations of heavy metals and pesticides in food waste that exceed limits allowed in conventional protein sources [Dou et al., 2018]. Establishing standards and schemes for the certification of treatment techniques and practices, and types of wastes that are appropriate for conversion to livestock feed may be required to avoid relevant food safety threats [Westendorf,, 2000]. Unmonitored and unregulated processing and feeding practices can lead to large disease outbreaks with severe consequences for the growth of the livestock sector, like the 2001 Foot-and-Mouth disease in the United Kingdom [Mens et al., 2021]. Such incidents further contribute to feelings of distrust by the consumers and lack of confidence in that regulatory authorities can guarantee the safety of novel feeds and consequently of livestock products.
Animal-based protein sources have been used primarily to counteract some of the drawbacks of plant-based feeds such as their relatively low protein content and presence of several anti-nutritional factors that reduce nutrient availability and tackle major sustainability issues such as land use and global warming potential [Lasekan, Bakar & Hashim, 2013; DiGiacomo & Leury, 2019]. While processed animal proteins (PAPs) of fish origin (i.e., fish meals) have been a common feed ingredient for many livestock species, in many countries legislation prohibits the use of PAPs from other species (e.g., avian PAPs) to prevent potential disease outbreaks like the epidemic of bovine / transmissible spongiform encephalopathies (BSE/TSE) in the 1980s [Lecrenier et al., 2020; Woodgate & Wilkinson, 2021]. Advancements in PAP rendering technologies and the controlling and regulatory processes may lead to lifting of such bans, as happened recently in the European Union (EU) [EU Commission, 2021]. The EU now allows for swine PAPs to be used in avian diets and vice versa, while insect PAPs can be used in either. However, ruminant PAPs are still prohibited to prevent a reoccurrence of TSE outbreaks, with the exception of milk, collagen and gelatine that can be used only for non-ruminants [Ricci et al., 2018].
Insects are also becoming increasingly popular both as potential feeds and human foods, with insect meals typically containing between 50 – 82% crude protein as well as other important nutrients (e.g., calcium, iron) [Madau et al., 2020]. As natural decomposers, insects can be reared in a great variety of substrates from feedstock material to waste, therefore converting unwanted substrates into sources of high-quality protein and healthy fats. To date, insect protein meals, mainly from five species have been explored and commercially exploited in bird and pet feeds, while their introduction in the livestock feed sector is a subject of further research [Manceron et al., 2014; Kim et al., 2019; van Huis et al., 2021].
Environmental implications
Land degradation, land use change and land availability related impacts
As a circular approach, using swine, poultry and ruminant PAPs in livestock feeds can help achieve similar overall environmental benefits to those discussed for protein obtained from food waste and industry waste streams, and cellular protein reared on waste. The production of insect protein for livestock feed at commercial scales however, presents slightly more complex interactions with the environment [Madau et al., 2020; van Huis et al., 2021].
Insect farming can potentially reduce land use requirements for protein production e.g., up to 98% when compared to soybean-fishmeal protein mixtures [van Huis & Oonincx, 2017]. However, it should be noted that insect farms (i.e., facilities) are not landless units and therefore unintended land-use impacts may become relevant for insect farming too as the sector expands i.e., with the displacement of other agricultural activities or driving land-use change in urban areas [Doi & Mulia, 2021].
Greenhouse gas emissions, atmospheric pollution and fossil fuel depletion
Mass rearing of insects for livestock feeds overall generates less GHG emissions when compared to conventional protein sources (e.g., fish meals, soy). However, it requires higher amounts of energy mainly for indoor climate regulation, an important condition for optimal insect growth as they are poikilotherms [van Huis & Oonincx, 2017; van Zanten et al., 2015]. Despite the high energy requirements, insect farming is not heavily dependent on fossil fuel because its typical farming operations involve the use of electricity. Therefore, there is a potential to fulfil these requirements through renewable energy sourcing and consequently further reduce the carbon footprint of livestock feed overall [Wang & Shelomi, 2017]. It is important to consider that insect farming is still at its infancy and there is a lot of room for improvement regarding resource efficiency, particularly considering the ability of insects to be reared on a wide range of substrates including various wastes [Bosch et al., 2019].
Nitrogen and phosphorus related impacts
In addition, rearing insects on manure produced by livestock (i.e., circular approach) has the potential to further reduce total nitrogen concentrations in agricultural soils by up to 62% [Elahi et al., 2022]. Further research should explore synergies and potential benefits from using different industry waste streams (e.g., food wastes) to identify optimal insect rearing strategies depending on specific regional agri-environmental policies; for example, organic wastes from livestock and households could be funnelled towards insect farming to help reduce overall concentrations of nitrogen and phosphorus in designated vulnerable zones (i.e., Nitrogen Vulnerable Zones, Phosphorus Vulnerable Zones) mitigating acidification and eutrophication impacts [Huygens et al., 2020].
Impacts to water quality and depletion of water resources
Although insects rely heavily on water for their growth, the overall water requirements for mass insect rearing are still significantly lower compared to conventional protein crop production [Wang & Shelomi, 2017]. The benefits of insect farming on depletion of water resources and water quality can be even greater if wastewater sludge and slurries are considered as substrates for rearing (i.e., upcycling of wastewaters to valuable protein). Under such scenarios, it is important to note that alternative uses of wastewater and their benefits can vary across geographies. For example, wastewater could be used for the production of energy, irrigation in conventional agriculture, or domestic (e.g., showering, washing) and non-domestic use (e.g., firefighting, swimming pools), all of which could be prioritised differently depending on countries’ water-stress levels [Zhao et al., 2019; Malone & Newton, 2020].
Impacts to biodiversity
As discussed for the cellular and circular agriculture alternatives above, adopting soilless protein production strategies such as protein from animal and insect PAPs can have several indirect positive implications for the conservation of global biodiversity, primarily through mitigating habitat loss, fragmentation and degradation impacts caused by conventional crop production [Jansson & Berggren, 2015]. However, it is important to consider that poorly managed mass rearing facilities may introduce risks for displacement of local species, i.e., through uncontrolled mixing of farmed and wild insect populations [Jansson & Berggren, 2015; van Huis et al., 2021].
Economic implications
Production and supply economics and resilience to extreme events
The economic feasibility of mass rearing of insects has been a topic of debate and conflict in recent literature. Due to high requirements for energy, the relatively low prices of competing conventional protein sources, and challenges in the marketability of insects as feed for livestock systems in Europe and Western countries, insect farming has not yet been exploited extensively at industrial scales [Arru et al., 2019]. On the other hand, insect farming can help avoid costs associated with synthetic and chemical inputs (i.e., does not require fertilisers), fossil fuel use (i.e., relies more on renewable energy), transportation (i.e., insect farm facilities can be located in or near urban areas) and labour [DiGiacomo & Leury, 2019; WWF, 2021]. Considering also the potential for insects to be reared on industry wastes (i.e., circular business model) further increases its potential cost-effectiveness through discounts in waste disposal of associated industries [Chia et al., 2019; Madau et al., 2020]. This diversity of potential synergies with other industries suggests that insect farming can be a resilient protein production system, robust to changes in availability and pricing of rearing substrates / inputs. Furthermore, its resilience can be enhanced with developments in the renewable energy sector that may enable uninterrupted and less expensive supply of electricity from renewable sources [DiGiacomo & Leury, 2019; WWF, 2021; van Huis et al., 2021].
Social implications
Nutritional value and animal growth
Insects are natural decomposers and great at converting energy embedded in organic matter into high concentrations of edible protein ranging between 35% and 82% [DiGiacomo & Leury, 2019; WWF, 2021]. In addition to this, insect meals provide livestock with important bioactive compounds and nutrients, such as iron, zinc, antimicrobial peptides, chitin, and lauric acid that can improve gut health and promote growth [Gasco et al., 2018; Madau et al., 2020]. Although evidence indicates that insects are a great source of high-quality protein for livestock, it is important to consider that their nutritional value may vary between insect species and depending on the substrate they are reared on [Oonincx & Finke, 2021; Pinotti & Ottoboni, 2021; WWF, 2021].
Animal health and welfare
While swine, poultry, and ruminant PAPs are a good source of protein and beneficial nutrients that promote animal growth and gut health, the way they are incorporated to livestock feeds should be carefully considered. Cross-feeding strategies appear to be critical in order to minimise the risks for viral contaminations that can cause significant impairments to animal health and welfare, or for severe diseases caused by prions such as BSE/TSEs.
As discussed in previous Chapters (i.e., protein production from cellular agriculture and food waste), using waste substrates for mass insect rearing may introduce important threats to their health and welfare, such as the accumulation of heavy metals, pesticides, microplastics and other contaminants that can reduce insect growth and increasing mortality rates [Schrögel & Wätjen, 2019]. An important issue to consider in relation to this is the ambiguity around the classification of farmed insects as feed or livestock. The latter could require strict standards to be followed in the insect farming industry to ensure insect health and welfare. Further, insects carrying biological and chemical contaminants are then fed to farmed fish/shellfish or livestock, and the contaminants reach their tissues where they cause various complications such as intestinal blockages, reducing animal appetite and growth.
Social development
Aside from promoting human wellbeing with the upcycling of animal by-products and wastes used for insect rearing, such alternatives present great opportunities for socio-economic growth. Due to the range of insect species that are suitable for consumption by animals, there can be economic, stable, locally produced insect farming solutions that allow smallholder livestock producers globally to be self-sufficient and avoid large costs associated with purchasing of conventional protein feed ingredients (Fig. 6) [Chia et al., 2019]. Furthermore, because insect farming is just starting to develop and considering its requirements for energy, hygienic treatment of waste substrates, and detection of microscopic contaminants, it may be a great driver for innovations in biotechnologies, regulatory and safety assessment systems and policies of the livestock sector, and the food-energy-water nexus overall [Ojha et al., 2021; Sindermann et al., 2021].
Figure 6: Contribution of insect farming to global Sustainable Development Goals from a socio-economic perspective. Source: Chia et al. (2019)
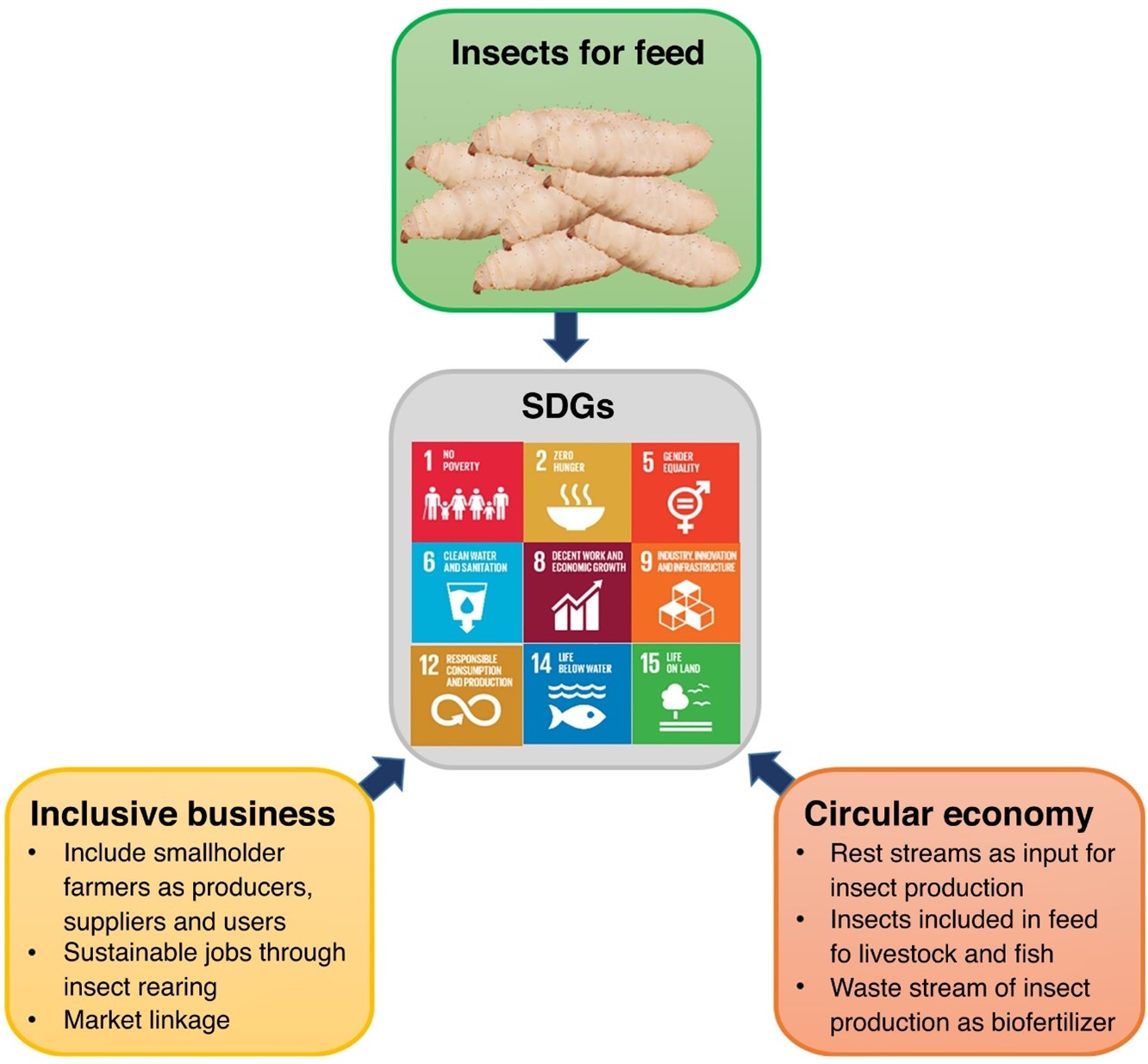
Consumer perception and acceptance
Along with legislation, consumer acceptance and attitudes towards the use of protein feeds from insects and animal by-products present the most important barriers to scale-up of these alternatives. There are major concerns regarding the extent to which religious and cultural factors would affect consumers acceptance of livestock reared on animal and insect PAPs, which could significantly limit the available markets. Such concerns mainly relate to the use of swine or beef PAPs that are considered forbidden in certain religions and cultures. Biases (e.g., disgust) developed through lack of understanding and socio-cultural misinformation significantly contribute to stakeholder distrust towards the safe and successful adoption of alternative proteins in livestock feeds. However, there are still gaps in research and more efforts are required to understand whether circular agriculture alternatives and insects are more acceptable when used as feeds rather than foods [van Huis et al., 2021]. In many cultures, including the Western world, insects reared for feed are viewed as livestock and therefore, consumers require insect rearing systems to adhere to high animal welfare standards. Retailer requirements and livestock certification standards could put further pressures on the growth and adoption rates of such protein feeds, requesting safety assurances to prevent food safety and mislabelling issues [van Huis et al., 2021; WWF, 2021].
Food safety
The re-introduction of PAPs from swine, poultry, and ruminants to livestock feeds raises some critical biosecurity concerns, mainly regarding past experiences of human disease outbreaks like the BSE/TSE epidemic of the 1980s [Woodgate & Wilkinson, 2021]. To minimise such risks, animal by-products and PAPs should be fed strictly cross-species (e.g., swine PAPs to poultry and vice-versa). Livestock feed producers that use PAPs from more than one species should carefully consider potential risks of cross-contamination and avoid mixing and milling such feeds using the same facilities [Lecrenier et al., 2020; Woodgate & Wilkinson, 2021].
Another major food safety risk arises with the mass rearing of insects, which can also be vectors of diseases and lead to pathogen contaminations threatening animal and eventually human health [van Huis et al., 2021]. When reared on livestock manures insects may carry veterinary drug residues, hormones and biological contaminants (e.g., bacteria with transgenic DNA, mycotoxins). When reared on plant and crop production waste, they may be exposed to pesticides and herbicides and mycotoxins. In addition, there is a risk for heavy metals and micro and nanoplastics to be accumulated into insects through waste substrates [Truzzi et al., 2020]. Bioaccumulation pathways then such as “animal feed è livestock è manure è farmed insects è livestock è humans”pose serious threats to human health and overall food safety. In addition to causing impairments in animal performance and welfare as discussed above, contaminants can enter the human food chain through livestock product consumption and cause severe effects like inflammatory responses, disruption on gut microbiota and effects on nutrient absorption, and even chronic inflammations that increase the risks for cancer [Smith et al., 2018; Prata et al., 2020]. The extent of bioaccumulation varies across the different farmed insect species, the growth phase they are in when exposed to contaminants, and the type of biological contaminant, metal or plastic particle [van der Fels‐Klerx et al., 2018; Meyer et al., 2021]. Thus far, studies have shown that the concentrations of both biological and chemical contaminants significantly decrease as insects reach adulthood [BfR, 2019; Schrögel & Wätjen, 2019]. However, more research is required to understand the specific pathways for the different contaminants and quantify bioaccumulation at each key step of these pathways up until human consumption (e.g., insect growth phases, animals digestive tract, livestock meat, milk, eggs) to better inform prevention strategies and regulatory systems [van der Fels‐Klerx et al., 2018]. To date, several mitigation strategies have been established to address the risk of biological contaminants and specifically the removal of pathogens at PAPs production phase. The “kill-step” is a well-used strategy in food safety that involves killing pathogens using thermal processing over time. Common thermal processing practices include pasteurisation, freezing, and heating / cooking. While these strategies are highly effective, there is a need to enhance our understanding of bioaccumulation pathways and processes that can potential change the physicochemical properties of both biological and chemical contaminants to further reduce the risks for food hazards.
Finally, allergens introduced to livestock and humans from insects may pose a serious risk for food safety. Studies have investigated the potential for cross-reactivity between edible insects and “domestic” insects and found that tropomyosin is such a cross-reactive allergen. They further investigated potential treatment methods to reduce allergenicity of edible insects and concluded that the reactivity of immunoglobulin is resistant to thermal processes and enzymic digestion. Therefore, evidence suggests that individuals allergic to “domestic” insects and those who are involved in insect farming should be considered as high-risk groups regarding their exposure to livestock products reared on insects or edible insects [Ribeiro et al., 2021].
Regulatory implications
Regulatory and safety assessment systems should employ state-of-the-art biotechnologies and practices to detect PAPs (e.g., specific immunoassay and polymerase chain reaction methods, infrared microscopy) and frequently reassess the prevalence of classical and atypical forms of BSE/TSEs [BIOHAZ, 2011].
To ensure safe mass rearing of insects for livestock feeds, thorough safety assessments and close monitoring of farming practices should be established, while pathogen resistant genotypes should be explored by future research (e.g., understanding of virome in commercially reared crickets) [de Miranda et al., 2021].
Table 2: Could insects be a sustainable protein source for livestock? The table summarises the main environmental, economic, and social impacts associated with the commercial implementation of insect meals as protein source for livestock feeds.
Environmental Implications
- Insect farming presents opportunities to combat critical environmental pressures. It does not rely on extensive land areas and fossil fuel inputs to the same extent as conventional protein crop production.
- It can be implemented in a wide range of climate conditions and locations, help reduce pressures on land and soil quality, and keep climate-related impacts to a minimum through the use of renewable resources.
- At larger scales it becomes a very energy demanding operation and so it should rely mostly on renewable energy rather than fossil fuel.
- Preliminary evidence points towards significantly lower impacts than conventional protein crop production for biodiversity, water quality, acidification, and eutrophication.
Economic Implications
- High start-up and operational costs make the exploitation of insect farming at industrial scales difficult.
- The lack of government financial support, private investments, and the competition for resources with subsidised industries create suboptimal conditions for growth of this production system, particularly in the absence of a stable market.
- Standardisation and assurance of production standards and inclusion limits may be required to encourage retailers to support livestock producers to use insect meals.
Social implications
- Insects can be reared on waste substrates, therefore upgrading them to healthy fats and proteins.
- Adding value to waste promotes a strong sense of caring for the environment.
- Their production relies less on manual labour than conventional protein sources and presents opportunities to promote innovation through automation of farming operations.
- There is a threat that insects can be disease vectors particularly if reared on waste, and that using insect processed proteins may lead to disease outbreaks.
- Strict legislation on waste substrates, insect processing, and feeding strategies are needed to minimise feed and food safety risks from insects as vectors of diseases.
- Further understanding agri-food stakeholders’ perspectives on insect farming is imperative to combating biases, misinformation, and to work towards silver bullet solutions.
Tables 3-6 below summarise the potential opportunities and risks to sustainability and food safety associated with the commercial implementation of the alternative protein ingredients discussed in this REA.
Table 3: Environmental opportunities and risks associated with the incorporation of alternative protein sources in livestock feeds
Environmental Implications | Opportunities | Risks |
Land degradation, land use and land availability related impacts |
Diversify and shift protein production from the global South reducing deforestation and land-use change pressures in endangered ecosystems (e.g., Amazon) – e.g., insect farming uses 90% less land compared to soy production. Soilless crop growing methods resulting to more land available for food production and healthier soils overall.
|
Poor management of former arable areas leading to abandoned and deserted lands. Geographical shift in protein production increasing feed-food competition for land in the global North and knock-on effects of land-use.
|
Greenhouse gas emissions, atmospheric pollution and fossil fuel depletion |
Reduced events of biomass burning and deforestation. More land occupied by trees leading to healthier atmosphere. Less synthetic and chemical inputs required for soilless and circular alternatives, therefore less energy required and emissions for their production. |
Specific operations of soilless alternatives (e.g., drying process at seaweed farming) may require large amounts of energy, which can increase fossil fuel depletion pressures unless renewably sourced. |
Impacts to biodiversity |
Reduced synthetic and chemical inputs improving conditions for terrestrial & aquatic life. Reduced events of biomass burning and deforestation leading healthier and less fragmented habitats and ecosystems. Seaweed farming creating new habitats for aquatic wildlife.
|
Invasiveness/weediness of GM/GE crops. Gene flow from GM/GEs threatening wild genotypes. Poor management of mass insect rearing facilities resulting to contamination of ecosystems with non-native species.
|
Nitrogen and phosphorus related impacts |
Less synthetic fertilisers for crop production leading to reduced leaching to soils and water, therefore reducing acidification & eutrophication pressures. Recycling of N and P from food waste, former foods, animal by-products, and industry by-products through circular agriculture, reducing nutrient leaching at waste disposal.
|
Freshwater algae and seaweed farming leading to increased N and P concentrations in aquatic ecosystems. Changes in manure composition of livestock due to consumption of alternatives, resulting to increased N and P deposition at field application or emissions at storage.
|
Impacts to water quality and depletion of water resources
|
Reduced requirements for irrigation due to more resilient GM/GE genotypes and soilless alternatives. Less synthetic and chemical inputs leading to healthier groundwater for human consumption. Upcycling of wastewater through circular and cellular agriculture. |
Increased water requirements for hydroponics, insect farming, and food waste processing at commercial scales. |
Table 4: Economic opportunities and risks associated with the incorporation of alternative protein sources in livestock feeds.
Economic Impacts | Opportunities | Risks |
Production and supply economics |
Reduced costs associated with synthetic and chemical inputs (e.g., fertilisers, pesticides, herbicides). Reduced costs for transportation and import of protein feeds from long distances / international, as well as easier access to labour with cellular and circular agriculture hubs or insect farms located closer to feed manufacturers and livestock production systems.
|
High capital / start-up costs for implementation of some soilless alternatives (e.g., seaweed, insect farming) at commercial scales. Reduced availability of novel technologies required for commercialisation. High operating costs due to energy requirements, especially as long as renewable energy prices remain high. High operating costs due to energy requirements, especially as long as renewable energy prices remain high.
|
Robustness to economic uncertainties and extreme events |
Protein production from GM/GE and local crops being more resilient to extreme climate and weather events and interruptions in supply chains (e.g., reduced losses due to long delays in transportation). Uninterrupted and robust to global trading dynamics supply by diversifying with soilless alternatives and shifting to increased local protein production. |
Sensitive to volatile energy prices due to high energy requirements. Cost-effective commercial implementation depending largely on advancements in biotechnology. |
Table 5: Social opportunities and risks associated with the incorporation of alternative protein sources in livestock feeds.
Social Implications | Opportunities | Risks |
Animal health and welfare |
Crude protein content as high as 93% (e.g., poultry PAPs) Good sources of fats and bioactive compounds that promote animal gut health and growth Animal by-products, insects, and food wastes characterised by enhanced bioavailability of nutrients compared to most plant-based protein sources Reduced risk for animal diseases (e.g., Foot and Mouth disease) caused by poor transportation and storage conditions of protein crops
|
Uncertainties around complete GM genome and functionality Acceptability by livestock of alternative and novel feeds resulting to inefficient feeding and impaired growth Poor hygienic processing in circular streams (e.g., food waste) resulting to accumulation of biological (e.g., mycotoxins) and chemical (e.g., nano-plastics) contaminants in livestock tissues
|
Social development |
Increased feed availability due to shorter supply chains Reduced heavy-duty on-farm labour Innovation in production / supply chains
|
Automation of production and supply chains for alternative proteins resulting to higher unemployment rates in agriculture Reduced protein feed production from the global South resulting to impoverishment of rural communities
|
Consumer perception and acceptance | Sustainable alternatives promote a “feel good” factor |
Lack of understanding and misinformation Increased incidents of feed fraud and mislabelling to make livestock products more appealing to consumers Some alternatives like insects or food waste may promote a “disgust” factor |
Table 6: Food safety related opportunities and risks associated with the incorporation of alternative protein sources in livestock feeds
Food safety | Opportunities | Risks |
Biological contamination | Less mycotoxin outbreaks caused by storage and transportation implications. |
Uncertainties around complete GM genome and functionality. Increased risk for viral disease outbreaks from animal by-products, such as BSE/TSEs. Poor processing of wastes for protein feed production resulting in increased risk for pathogens to reach humans.
|
Chemical contamination | Less pesticides / uptake of heavy metals from irrigation waters. | Poor processing of wastes for protein feed production resulting in increased risk for contamination of humans by microplastics, nano-plastics, and packaging remnants through bioaccumulation. |
Allergenicity | GM/GE crop variants potentially reducing the expression of proteins causing allergies. | Major allergens introduced in human foods through bioaccumulation from several alternative feeds such as GM/GE crops, legumes, seaweed, and food waste. |
Implications and assessment of sustainability trade-offs
The sustainability of livestock feed production is an inherently complex concept that involves the management of available land and resources, considering their optimal use for multiple objectives [Nyström et al., 2019]. The REA identifies sustainability trade-offs as the situations in which achieving a good performance in one of these objectives comes at the expense of the performance in another. Sustainability trade-offs can be found across all different hierarchical levels of the sector, from the protein crops, the farm and farming business, to the landscape and the markets. For example, shifting to landless cultivations (e.g., hydroponics, aquaculture) or moving much of protein feed production to the global North can alleviate environmental pressures of the global South and free-up land, however it may exacerbate poverty and social exclusion of south rural communities. Adopting circular agriculture alternatives (e.g., protein feed from former foods, food waste, by-products) can significantly reduce the environmental footprint of livestock feeds but can also potentially lead to large instabilities in the global agri-food market, reveal new risks for food security, and even lead to production sub-systems and markets dedicated to by-products that may generate further impacts. Overall, how can we ensure that the socio-economic gap created by the adoption of alternative protein feeds, will not be filled with unsustainable practices potentially resulting to even larger negative impacts than what conventional protein feed production generated?
Such trade-offs become particularly prominent when stakeholder objectives conflict and when there are limits on available resources to achieve sector goals (e.g., economic restrictions, issues of land availability) [Patterson et al., 2017]. Within- and across sustainability pillars trade-offs are ubiquitous in the decision-making of future alternative protein feeds. The REA proposes that all three sustainability pillars are considered equally, to enable stakeholders of the livestock sector including policy makers, farm managers, and researchers to identify and evaluate key trade-offs for the sustainable commercialisation of alternative protein feeds (Table 7).
For the assessment of such trade-offs, stakeholders need to adopt a holistic approach when evaluating agri-food system performance [Green et al., 2020]. Simulation and optimisation life cycle assessment (LCA) models, and multi-criteria decision analyses (MCDA) quantitatively evaluate the sustainability impacts associated with the various inputs and outputs of alternative protein feed production. However, because most of these alternatives have not yet been adopted at commercial scales, in combinations, and in diverse livestock systems, empirical research should generate more detailed datasets to reduce uncertainties and enhance understanding of livestock protein scenarios. Furthermore, there are no sets of reference scenarios to describe a range of plausible socio-economic futures for the agri-food sector, unlike what the global climate modelling community has established, which makes predictions even harder [Rosenzweig et al., 2016]. Another inherent limitation of these models is that agri-food stakeholders often do not behave predictably and according to economic, social or environmental rationality. For example, farmers may not necessarily invest large amounts of capital in a green technology (e.g., anaerobic digestion) even if projections show increased returns on investment and improved environmental performance [Klapwijk, et al., 2014]. Participatory approaches (e.g., interactive cognitive mapping), interviews and focus groups could help understand stakeholder behaviour, knowledge, and perception better, offering critical information for the implementation of alternative protein feeds. Regardless of developments in quantitative trade-off assessments, the final interpretation of outputs is usually left with the stakeholders and so it is imperative that their needs, concerns and vested interests are well understood to ensure that sustainability solutions are realised [Brick et al., 2018; Journeault et al., 2021]. Stakeholder objectives often change in time and with geography determining which trade-offs are more relevant, which should be considered when working towards the future of livestock feeds [Kanter et al., 2018].
Table 7: Examples of sustainability trade-offs in using alternative protein sources for livestock feeds. The table presents recommendations for focus of future research and key stakeholders for participation in a dialogue for the better understanding of key sustainability trade-offs in the livestock feed sector.
Trade-off | Future research recommendations | Stakeholders' dialogue |
Reducing land use with soilless feed alternatives frees arable land for food production and helps conserve wildlife habitats. However, if not properly managed unoccupied land can degrade quickly and lead to more fragmented habitats. | Better biodiversity indices, improved soil horizon scanning & soil organic carbon monitoring techniques. | Farmers, policy makers, local communities |
Pest resistant GM/GE protein crops require less pesticides/herbicides leading to less chemical pollution for agricultural biodiversity. However GM genotypes they may threaten wild types due to gene transferring or displace local species. | Better understanding of GM genomes and interactions with wild types. | Researchers, farmers, policy makers, local communities |
Using waste streams as substrates in cellular agriculture or insect farming reduces costs of production and avoids costs for waste disposal. However, it raises biosecurity concerns due to increased potential for bioaccumulation of biological and chemical contaminants from waste to human foods. | Advancements in biotechnology for better understanding of bioaccumulation pathways and detection of nanoparticles / contaminants. | Researchers, policy makers, consumers |
Protein feeds from cellular and circular agriculture reduce industrial waste. However, they raise risks for consumer acceptability and therefore, jeopardise market viability of livestock products. | More research on relevant consumer perception and attitudes, cultural and religious barriers, retailers and industry stakeholder requirements and concerns. | Local communities, consumers, farm managers, retailers |
Cellular and circular alternatives promote innovation in the agri-food sector leading to the development of automated, resource-efficient protein production and supply chains. However, they may have increased requirements for expensive technologies and specialised labour, which may drive smallholder feed producers out of the competition and increase unemployment. | More quantitative and accurate modelling of the socio-economic implications of large scale cellular and circular agriculture under various macro-economic scenarios | Farm managers, policy makers, local communities, retailers, consumers |
Emerging threats within and beyond a 5-year horizon
Livestock sector stakeholders need to consider several emerging threats. Reviewing recent scientific and grey literature, the REA identifies significant socio-economic threats in the immediate and intermediate short-term (now to the next five years), due to the on-going global energy crisis, and the increased volatility of fuel and agricultural commodity prices causing instabilities in global trading. The Ukraine-Russia conflict has blocked the supply of sunflower meal from one of the largest producers globally (Ukraine) and has frozen large European investments that aimed to support Ukrainian soy production replacing unsustainable imports. Other recent geo-political developments like Brexit have exacerbated feelings of insecurity and distrust of agricultural stakeholders, as well as created concerns and instabilities in future trading partners, supply of labour, import/duty policies, agri-environmental schemes and new requirements to receive support through subsidies [Grant, 2016; Swinbank, 2017; Chang, 2018]. Political conflicts have caused significant delays in protein feed trading (e.g., delays at Ukrainian ports due to Ukraine-Russia conflict), therefore increasing the risks for biological contamination (e.g., aflatoxin) due to poor storage and transportation conditions [Zupaniec et al., 2021]. Potential disease outbreaks threatening feed and food security should also be considered with the reintroduction of PAPs for livestock feeds in Europe.
In the longer time horizon (beyond five years), climate-related impacts on global protein feed production are expected. Anticipated threats include impaired productivity and poorer nutrient profiles of conventional protein feeds, due to extreme and damaging climate-events (e.g., droughts, frost, hail) and soil degradation i.e., poor soil organic horizons due to intense agricultural activity and prolonged droughts. Finally, climate change-contaminant interactions, such as increase in mycotoxin contamination due to increased feed ingredient moisture, are expected to lead to increased outbreaks of biological contaminants and alternations in the pathways of bioaccumulation [Alava et al., 2017].
Roadmap for future research and discussions
Like any other extensive evidence assessment, this report acknowledges specific limitations which highlight potential directions for future research and discussions. The REA captures most of the environmental, economic and social implications that are immediately relevant to FSA and the UK but are also important for global sustainable development of the livestock feed sector. It focuses on the most mature, well-established and well-explored alternative protein feed solutions as these have been identified through scientific literature, governmental reports and expert opinion. However, it acknowledges that there may be other less-known, currently under development, or yet unrecognised potential alternatives, which the REA does not consider primarily due to limitations in availability of relevant data and information. Such limitations make the discussion regarding suitability of potential alternatives at commercial scales particularly difficult. Furthermore, the REA recognises that considering the rapid advancements in the energy sector and biotechnologies, and the uncertainties in macro-economic and geo-political developments, additional sustainability implications may arise with the future implementation of these solutions at industrial scales.
Another important limitation to consider is that this report focused specifically on the use of alternative protein sources as ingredients for livestock feed; however, there may be important implications for their economic viability and overall sustainability that can be explored through a more holistic approach that considers their specific interactions with the human food chain. The report acknowledges also that while it identified key sustainability trade-offs for the implementation of alternative proteins at commercial scales, more in-depth research is required to better understand and quantitatively evaluate them under various spatiotemporal scales.
Key recommendations for policy making
The REA synthesises four broad directions for policy making and research that may enable the potential contribution of alternative protein feeds to global sustainable development goals (Table 8) [UNDESA, 2022].
- Decoupling protein production from fossil fuel should be the first focus of policies and action in the livestock feed sector. Replacing diesel and gas with energy from renewable resources can reduce the carbon footprint of the livestock sector overall. Renewable energy prices should be regulated to ensure feed market stability, and feed producers and manufacturers should have access to energy from multiple renewable sources (e.g., solar, wind, geothermal) to allow for abundant and uninterrupted supply. This may enable sustainable adoption and unlock the full potential of alternative protein feeds that require large amounts of energy for feed processing (e.g., insect meals, food waste, former foods).
- Developing sustainable economic strategies for alternative proteins at a subnational level can help relieve great amounts of environmental pressure particularly from areas that experience issues of deforestation, land degradation, and land availability, but also a large part of the carbon footprint that is associated with transportation of feed over long distances. Local feed prices may increase due to higher labour and other input costs, but the markets should be regulated to ensure that such increases do not outweigh economic benefits from avoided import duties and transportation costs. Local solutions may need to be financially supported to avoid livestock producers turning to less expensive imported alternatives. While an economic growth of local feed markets in the North may lead to overall socio-economic development of rural communities, there may be an opposite, degrading effect for areas of the South that will lose their production (e.g., the Brazilian Cerrado), which policies should consider.
- Supporting circular livestock feed solutions such as protein from food waste, former foods, animal by-products, and industry by-products, can help reduce land-related impacts, economic costs of crop production, and tackle food waste. This requires addressing the main obstacles of customer and producer acceptability through more efficient stakeholder engagement, understanding of their concerns, and clarifying relevant misinformation and biases. Educating and supporting on-farm labour, livestock producers, and consumers with matters of feed and food security that may arise from the adoption of circular alternatives can facilitate uptake of these sustainable alternative protein sources.
- Further enhancing the feed and food regulatory system with research on more sensitive early detection and monitoring methods for feed and food security risks. This is imperative for enabling the safe adoption of alternatives like cellular and insect protein reared on waste substrates, food waste and former foods as protein sources, and processed animal proteins. Emerging feed and food security threats, like the impacts of climate change and storage/transportation conditions on biological contaminant blooms, should be considered throughout.
- These recommendations are not mutually exclusive and propose a roadmap and research agenda towards a more sustainable livestock feed production and achieving several global sustainable development goals. Immediate action is required in the coming years in reshaping the global livestock feed market to enhance its resilience against macro-economic and geopolitical instabilities (e.g., war in Ukraine, energy crisis, Covid-19 restrictions). Potential interactions between sustainable feed solutions and trade-offs within and between sustainability pillars, should be further researched to identify impacts on stakeholders across spatiotemporal scales. Anticipatory policies should be in place to compensate for losses through such trade-offs and to scope the future of the livestock sector beyond the time horizon suggested by the current sustainability agenda (i.e., 2030 as in UN SDGs). New research should adopt more transdisciplinary and co-design approaches to map the stakeholder power and potential to enable sustainable solutions for the livestock feed sector and get a better insight into the complexities of the less understood socio-economic implications. In addition, future research should investigate how the capacity of land to accommodate the production of any alternative protein sources, including the ones discussed here, changes under different demand scenarios [Shah & Wu, 2019].
Table 8: Example of interactions between alternative protein sources for livestock feeds and global sustainable goals
Sustainability goals | Mechanism |
Socio-economic resilience against climate-related, macroeconomic, and geo-political extreme events (SDG 1) | Decoupling protein feed production from fossil fuel and economically volatile energy sources; reduced reliance on imported protein and global trading partnerships |
Increase food security and end hunger (SDG 2) | Landless protein sources reducing feed-food competition; increasing protein feed availability and improving accessibility through local markets; reduced protein feed costs through the use of circular agriculture alternatives leading to less expensive / accessible livestock products |
Improve water quality and water-use efficiency, supporting the participation of local communities in water security (SDG 6) | Reduced reliance on groundwater resources for irrigation; reduced chemical pollution of water bodies by avoiding synthetic fertilisers / chemical inputs at crop production |
Promote job creation, and safe and secure working environments (SDG 8) | More diverse labour input requirements; reduced heavy-duty manual labour compared to conventional crop production |
Resilience and adaptive capacity to climate-related hazards (SDG 13) & Carbon Net Zero emissions | Reduced reliance on fossil fuel, more land available for trees; healthier soil organic carbon stocks; reducing pressure on water cycle through reduced irrigation |
Nitrogen (N) and Phosphorus (P) Vulnerable zones to reduce eutrophication pressures | Reduced use of synthetic N and P fertilisers reducing nutrient leaching; reduced organic material deposition in water bodies due to healthier / more stable soils |
Minimise impacts of ocean acidification (SDG 14) | Reduced nitrogen leaching from soils due to the use of synthetic fertiliser |
Ensure the conservation and sustainable use of terrestrial and inland freshwater ecosystems and their services (SDG 15) | Healthier soil horizons; reduced potential for acidification of ecosystems; reduced impacts of habitat fragmentation and degradation for terrestrial and aquatic biodiversity |
Combat desertification, land and soil degradation, deforestation (SDG 15) | Reduced land requirements for protein crop production; reduced reliance on protein sources from environmental hotspots |
Reducing food waste, carbon, and protecting critical water resources (Courtauld Commitment 2030) | Food waste used directly as feed or substrate; reduced fossil fuel use leading to reduced carbon emissions; landless alternatives using significantly less water |
Makkar, H. P. S. (2018). Feed demand landscape and implications of food-not feed strategy for food security and climate change. Animal, 12(8), 1744-1754. https://doi.org/10.1017/S175173111700324X
Gurgel, A. C., Reilly, J., & Blanc, E. (2021). Challenges in simulating economic effects of climate change on global agricultural markets. Climatic Change, 166(3), 1-21. https://doi.org/10.1007/s10584-021-03119-8
Food and Agriculture Organization (FAO). (2019). Global Food Outlook November 2019 / FAO forecast. Available at: http://www.fao.org/news/story/en/item/1247138/icode/. Accessed on 15 December 2021.
Food and Agriculture Organization (FAO). (2018). World Livestock: Transforming the livestock sector through the Sustainable Development Goals. Rome. 222 pp. https://doi.org/10.4060/ca1201en
Food and Agriculture Organization (FAO). (2017). Animal Production and Health Division and the Livestock Environmental Assessment and Performance Partnership (LEAP). Available at: http://www.fao.org/partnerships/leap/en/. Accessed on 15 December 2021
Semper-Pascual, A., Decarre, J., Baumann, M., Busso, J. M., Camino, M., Gómez-Valencia, B., & Kuemmerle, T. (2019). Biodiversity loss in deforestation frontiers: linking occupancy modelling and physiological stress indicators to understand local extinctions. Biological Conservation, 236, 281-288. https://doi.org/10.1016/j.biocon.2019.05.050
Adam, M. G., Tran, P. T., Bolan, N., & Balasubramanian, R. (2021). Biomass burning-derived airborne particulate matter in Southeast Asia: A critical review. Journal of Hazardous Materials, 407, 124760. https://doi.org/10.1016/j.jhazmat.2020.124760
Andretta, I., Hickmann, F. M., Remus, A., Franceschi, C. H., Mariani, A. B., Orso, C., Kipper, M., Létourneau-Montminy, M. P., & Pomar, C. (2021). Environmental Impacts of Pig and Poultry Production: Insights From a Systematic Review. Frontiers in Veterinary Science, 1232. https://doi.org/10.3389/fvets.2021.750733
van Huis, A., & Oonincx, D. G. (2017). The environmental sustainability of insects as food and feed. A review. Agronomy for Sustainable Development, 37(5), 1-14. https://doi.org/10.1007/s13593-017-0452-8
van Hal, O., Weijenberg, A. A. A., De Boer, I. J. M., & Van Zanten, H. H. E. (2019). Accounting for feed-food competition in environmental impact assessment: Towards a resource efficient food-system. Journal of Cleaner Production, 240, 118241. https://doi.org/10.1016/j.jclepro.2019.118241
Te Pas, M. F., Veldkamp, T., de Haas, Y., Bannink, A., & Ellen, E. D. (2021). Adaptation of livestock to new diets using feed components without competition with human edible protein sources—a review of the possibilities and recommendations. Animals, 11(8), 2293. https://doi.org/10.3390/ani11082293
Muscat, A., de Olde, E. M., de Boer, I. J., & Ripoll-Bosch, R. (2020). The battle for biomass: A systematic review of food-feed-fuel competition. Global Food Security, 25, 100330. https://doi.org/10.1016/j.gfs.2019.100330
Watson, C. A., Reckling, M., Preissel, S., Bachinger, J., Bergkvist, G., Kuhlman, T., Lindström, K., Nemecek, T., Topp, C. F. E., Vanhatalo, A., Zander, P., Murphy-Bokern, D., & Stoddard, F. L. (2017). Grain legume production and use in European agricultural systems. Advances in Agronomy, 144, 235-303. https://doi.org/10.1016/bs.agron.2017.03.003
Sherasia, P. L., Garg, M. R., & Bhanderi, B. M. (2018). Pulses and their by-products as animal feed. United Nations. Rome. 222 pp. ISBN: 978-92-5-109915-5
Sońta, M., Rekiel, A., & Batorska, M. (2019). Use of duckweed (Lemna L.) in sustainable livestock production and aquaculture–a review. Annals of Animal Science, 19(2), 257-271. https://doi.org/10.2478/aoas-2018-0048
Costa, M., Cardoso, C., Afonso, C., Bandarra, N. M., & Prates, J. A. (2021). Current knowledge and future perspectives of the use of seaweeds for livestock production and meat quality: a systematic review. Journal of Animal Physiology and Animal Nutrition, 105(6), 1075-1102. https://doi.org/10.1111/jpn.13509
Duarte, C. M., Bruhn, A., & Krause-Jensen, D. (2021). A seaweed aquaculture imperative to meet global sustainability targets. Nature Sustainability, 1-9. https://doi.org/10.1038/s41893-021-00773-9
Bartelme, R. P., Oyserman, B. O., Blom, J. E., Sepulveda-Villet, O. J., & Newton, R. J. (2018). Stripping away the soil: plant growth promoting microbiology opportunities in aquaponics. Frontiers in Microbiology, 9, 8. https://doi.org/10.3389/fmicb.2018.00008
van Eenennaam, A. L., & Young, A. E. (2014). Prevalence and impacts of genetically engineered feedstuffs on livestock populations. Journal of Animal Science, 92(10), 4255-4278. https://doi.org/10.2527/jas.2014-8124
Flachowsky, G., Chesson, A., & Aulrich, K. (2005). Animal nutrition with feeds from genetically modified plants. Archives of Animal Nutrition, 59(1), 1-40. https://doi.org/10.1080/17450390512331342368
Eriksson, M., Ghosh, R., Hansson, E., Basnet, S., & Lagerkvist, C. J. (2018). Environmental consequences of introducing genetically modified soy feed in Sweden. Journal of Cleaner Production, 176, 46-53. https://doi.org/10.1016/j.jclepro.2017.12.113
Gocht, A., Consmüller, N., Thom, F., & Grethe, H. (2021). Economic and environmental consequences of the ECJ genome editing judgment in agriculture. Agronomy, 11(6), 1212. https://doi.org/10.3390/agronomy11061212
Jones, S. W., Karpol, A., Friedman, S., Maru, B. T., & Tracy, B. P. (2020). Recent advances in single cell protein use as a feed ingredient in aquaculture. Current Opinion in Biotechnology, 61, 189-197. https://doi.org/10.1016/j.copbio.2019.12.026
Tropea, A., Ferracane, A., Albergamo, A., Potortì, A. G., Lo Turco, V., & Di Bella, G. (2022). Single cell protein production through multi food-waste substrate fermentation. Fermentation, 8(3), 91. https://doi.org/10.3390/fermentation8030091
Puyol, D., Batstone, D. J., Hülsen, T., Astals, S., Peces, M., & Krömer, J. O. (2017). Resource recovery from wastewater by biological technologies: opportunities, challenges, and prospects. Frontiers in Microbiology, 7, 2106. https://doi.org/10.3389/fmicb.2016.02106
Ritala, A., Häkkinen, S. T., Toivari, M., & Wiebe, M. G. (2017). Single cell protein—state-of-the-art, industrial landscape and patents 2001–2016. Frontiers in Microbiology, 8, 2009. https://doi.org/10.3389/fmicb.2017.02009
Nyyssölä, A., Suhonen, A., Ritala, A., & Oksman-Caldentey, K. M. (2022). The role of single cell protein in cellular agriculture. Current Opinion in Biotechnology, 75, 102686. https://doi.org/10.1016/j.copbio.2022.102686
Stephens, N., & Ellis, M. (2020). Cellular agriculture in the UK: a review. Wellcome Open Research, 5. https://doi.org/10.12688/wellcomeopenres.15685.2
Mattick, C. S. (2018). Cellular agriculture: the coming revolution in food production. Bulletin of the Atomic Scientists, 74(1), 32-35. https://doi.org/10.1080/00963402.2017.1413059
Hardy, R. W., Patro, B., Pujol‐Baxley, C., Marx, C. J., & Feinberg, L. (2018). Partial replacement of soybean meal with Methylobacterium extorquens single‐cell protein in feeds for rainbow trout (Oncorhynchus mykiss Walbaum). Aquaculture Research, 49(6), 2218-2224. https://doi.org/10.1111/are.13678
Glencross, B. D., Huyben, D., & Schrama, J. W. (2020). The application of single-cell ingredients in aquaculture feeds—a review. Fishes, 5(3), 22. https://doi.org/10.3390/fishes5030022
Pignolet, O., Jubeau, S., Vaca-Garcia, C., & Michaud, P. (2013). Highly valuable microalgae: biochemical and topological aspects. Journal of Industrial Microbiology and Biotechnology, 40(8), 781-796. https://doi.org/10.1007/s10295-013-1281-7
Helliwell, R., & Burton, R. J. (2021). The promised land? Exploring the future visions and narrative silences of cellular agriculture in news and industry media. Journal of Rural Studies, 84, 180-191. https://doi.org/10.1016/j.jrurstud.2021.04.002
Bapat, S., Koranne, V., Shakelly, N., Huang, A., Sealy, M., Sutherland, J. W., Rajurkar, K. P., & Malshe, A. P. (2021). Cellular agriculture: An outlook on smart and resilient food agriculture manufacturing. ASTM Smart and Sustainable Manufacturing Systems. https://doi.org/10.1520/SSMS20210020
Eibl, R., Senn, Y., Gubser, G., Jossen, V., van den Bos, C., & Eibl, D. (2021). Cellular agriculture: Opportunities and challenges. Annual Review of Food Science and Technology, 12, 51-73. https://doi.org/10.1146/annurev-food-063020-123940
Odegard, I., & Sinke, P. (2021). LCA of cultivated meat. Future projections for different scenarios. CE Delft, February, 22-55. Available at https://cedelft.eu/publications/tea-of-cultivated-meat/
Saavoss, M. (2019). How might cellular agriculture impact the livestock, dairy, and poultry industries?. Choices, 34(1), 1-6. https://www.jstor.org/stable/26758666
Gasteratos, K. (2019). 90 Reasons to consider cellular agriculture. Available at https://dash.harvard.edu/handle/1/38573490
Behm, K., Nappa, M., Aro, N., Welman, A., Ledgard, S., Suomalainen, M., & Hill, J. (2022). Comparison of carbon footprint and water scarcity footprint of milk protein produced by cellular agriculture and the dairy industry. The International Journal of Life Cycle Assessment, 27, 1017-1034. https://doi.org/10.1007/s11367-022-02087-0
Moritz, J., Tuomisto, H. L., & Ryynänen, T. (2022). The transformative innovation potential of cellular agriculture: Political and policy stakeholders’ perceptions of cultured meat in Germany. Journal of Rural Studies, 89, 54-65. https://doi.org/10.1016/j.jrurstud.2021.11.018
Teng, T. S., Chin, Y. L., Chai, K. F., & Chen, W. N. (2021). Fermentation for future food systems: Precision fermentation can complement the scope and applications of traditional fermentation. EMBO reports, 22(5), e52680. https://doi.org/10.15252/embr.202152680
Mainardes, G. A., & DeVries, T. J. (2016). Effect of social feeding environment on the feeding behaviour of dairy cows and their willingness to consume a novel feed. Applied Animal Behaviour Science, 185, 23-29. https://doi.org/10.1016/j.applanim.2016.10.002
Kuhad, R. C., Singh, A., Tripathi, K. K., Saxena, R. K., & Eriksson, K. E. L. (1997). Microorganisms as an alternative source of protein. Nutrition reviews, 55(3), 65-75. https://doi.org/10.1111/j.1753-4887.1997.tb01599.x
Williams, R. A. (2021). Opportunities and challenges for the introduction of new food proteins. Annual Review of Food Science and Technology, 12, 75-91. https://doi.org/10.1146/annurev-food-061220-012838
Zollman Thomas, O., & Bryant, C. (2021). Don't Have a Cow, Man: Consumer Acceptance of Animal-Free Dairy Products in Five Countries. Frontiers in Sustainable Food Systems, 223. https://doi.org/10.3389/fsufs.2021.678491
Tavill, G. (2020). Industry challenges and approaches to food waste. Physiology & behavior, 223, 112993. https://doi.org/10.1016/j.physbeh.2020.112993
Dou, Z., Toth, J. D., & Westendorf, M. L. (2018). Food waste for livestock feeding: Feasibility, safety, and sustainability implications. Global food security, 17, 154-161. https://doi.org/10.1016/j.gfs.2017.12.003
Luciano, A., Tretola, M., Ottoboni, M., Baldi, A., Cattaneo, D., & Pinotti, L. (2020). Potentials and challenges of former food products (food leftover) as alternative feed ingredients. Animals, 10(1), 125. https://doi.org/10.3390/ani10010125
Pinotti, L., Luciano, A., Ottoboni, M., Manoni, M., Ferrari, L., Marchis, D., & Tretola, M. (2021). Recycling food leftovers in feed as opportunity to increase the sustainability of livestock production. Journal of Cleaner Production, 294, 126290. https://doi.org/10.1016/j.jclepro.2021.126290
Rajeh, C., Saoud, I. P., Kharroubi, S., Naalbandian, S., & Abiad, M. G. (2021). Food loss and food waste recovery as animal feed: a systematic review. Journal of Material Cycles and Waste Management, 23, 1–17. https://doi.org/10.1007/s10163-020-01102-6
European Commission. (2015a). Closing the Loop-An EU Action Plan for the Circular Economy. Brussels: European Commission. Available at https://www.eea.europa.eu/policy-documents/com-2015-0614-final
Popescu, M. F. (2019). Is Circular Economy Going to Reduce Waste and Create Jobs in the European Union?. Economic and Social Development: Book of Proceedings, 398-406.
Jagtap, S., Garcia-Garcia, G., Duong, L., Swainson, M., & Martindale, W. (2021). Codesign of food system and circular economy approaches for the development of livestock feeds from insect larvae. Foods, 10(8), 1701. https://doi.org/10.3390/foods10081701
Zhu, Q., Jia, R., & Lin, X. (2019). Building sustainable circular agriculture in China: economic viability and entrepreneurship. Management Decision, 57(4), 1108-1122. https://doi.org/10.1108/MD-06-2018-0639
Salami, S. A., Luciano, G., O'Grady, M. N., Biondi, L., Newbold, C. J., Kerry, J. P., & Priolo, A. (2019). Sustainability of feeding plant by-products: A review of the implications for ruminant meat production. Animal Feed Science and Technology, 251, 37-55. https://doi.org/10.1016/j.anifeedsci.2019.02.006
Li, Y., Zhang, G. N., Xu, H. J., Zhou, S., Dou, X. J., Lin, C., Xing-Yi, Z., Hong-Bo, Z., & Zhang, Y. G. (2019). Effects of replacing alfalfa hay with Moringa oleifera leaves and peduncles on intake, digestibility, and rumen fermentation in dairy cows. Livestock Science, 220, 211-216. https://doi.org/10.1016/j.livsci.2019.01.005
WRAP. (2016). Using surplus food in animal feed. Available at: https://wrap.org.uk/resources/tool/using-surplus-food-animal-feed. Accessed on 1 March 2022.
Tallentire, C. W., Mackenzie, S. G., & Kyriazakis, I. (2018). Can novel ingredients replace soybeans and reduce the environmental burdens of European livestock systems in the future?. Journal of Cleaner Production, 187, 338-347. https://doi.org/10.1016/j.jclepro.2018.03.212
de Souza, N. R. D., Junqueira, T. L., & Cavalett, O. (2021). Opportunities and challenges for bioenergy-livestock integrated systems in
Brazil. Industrial Crops and Products, 173, 114091. https://doi.org/10.1016/j.indcrop.2021.114091
Stadtlander, T., Förster, S., Rosskothen, D., & Leiber, F. (2019). Slurry-grown duckweed (Spirodela polyrhiza) as a means to recycle nitrogen into feed for rainbow trout fry. Journal of Cleaner Production, 228, 86-93. https://doi.org/10.1016/j.jclepro.2019.04.196
Sońta, M., Łozicki, A., Szymańska, M., Sosulski, T., Szara, E., Wąs, A., van Pruissen, G. W. P., & Cornelissen, R. L. (2020). Duckweed from a Biorefinery System: Nutrient Recovery Efficiency and Forage Value. Energies, 13(20), 5261. https://doi.org/10.3390/en13205261
Lasekan, A., Bakar, F. A., & Hashim, D. (2013). Potential of chicken by-products as sources of useful biological resources. Waste Management, 33(3), 552-565. https://doi.org/10.1016/j.wasman.2012.08.001
DiGiacomo, K., & Leury, B. J. (2019). Insect meal: a future source of protein feed for pigs?. Animal, 13(12), 3022-3030. https://doi.org/10.1017/S1751731119001873
Woodgate, S. L., & Wilkinson, R. G. (2021). The role of rendering in relation to the bovine spongiform encephalopathy epidemic, the development of EU animal by‐product legislation and the reintroduction of rendered products into animal feeds. Annals of Applied Biology, 178(3), 430-441. https://doi.org/10.1111/aab.12676
EFSA Panel on Biological Hazards (BIOHAZ). (2011). Scientific Opinion on the revision of the quantitative risk assessment (QRA) of the BSE risk posed by processed animal proteins (PAPs). EFSA Journal, 9(1), 1947. Available at https://efsa.onlinelibrary.wiley.com/doi/epdf/10.2903/j.efsa.2011.1947
Lecrenier, M. C., Veys, P., Fumière, O., Berben, G., Saegerman, C., & Baeten, V. (2020). Official feed control linked to the detection of animal byproducts: Past, present, and future. Journal of Agricultural and Food Chemistry, 68(31), 8093-8103. https://doi.org/10.1021/acs.jafc.0c02718
van der Fels‐Klerx, H. J., Camenzuli, L., Belluco, S., Meijer, N., & Ricci, A. (2018). Food safety issues related to uses of insects for feeds and foods. Comprehensive Reviews in Food Science and Food Safety, 17(5), 1172-1183. https://doi.org/10.1111/1541-4337.12385
Ribeiro, J. C., Sousa-Pinto, B., Fonseca, J., Fonseca, S. C., & Cunha, L. M. (2021). Edible insects and food safety: allergy. Journal of Insects as Food and Feed, 7(5), 833-847. https://doi.org/10.3920/JIFF2020.0065
Meyer, A. M., Meijer, N., Hoek-Van den Hil, E. F., & Van der Fels-Klerx, H. J. (2021). Chemical food safety hazards of insects reared for food and feed. Journal of Insects as Food and Feed, 7(5), 823-831. https://doi.org/10.3920/JIFF2020.0085
German Federal Institute for Risk Assessment (BfR), National Reference Laboratory for Animal protein in Feed, NRL‐AP, Garino, C., Zagon, J., & Braeuning, A. (2019). Insects in food and feed–allergenicity risk assessment and analytical detection. EFSA Journal, 17, e170907. https://doi.org/10.2903/j.efsa.2019.e170907
‘Commission Regulation (EU) 2021/1372. (17 August 2021). Amending Annex IV to Regulation (EC) No 999/2001 of the European Parliament and of the Council as regards the prohibition to feed non-ruminant farmed animals, other than fur animals, with protein derived from animal.’ Official Journal L295(64). Available at: https://eur-lex.europa.eu/oj/direct-access.html. Accessed on 15 December 2021
Ricci, A., Allende, A., Bolton, D., Chemaly, M., Davies, R., Escámez, P. S. F., Gironés, R., Herman, L., Koutsoumanis, K., Lindqvist, R., Nørrung, B., Robertson, L., Ru, G., Sanaa, M., Skandamis, P., Snary, E., Speybroeck, N., Ter Kuile, B., Threlfall, J., Wahlström, H., Adkin, A., Greiner, M., Marchis, D., Prado, M., Da Silva Felicio, T., Ortiz-Pelaez, A., & Simmons, M. (2018). Updated quantitative risk assessment (QRA) of the BSE risk posed by processed animal protein (PAP). EFSA Journal, 16(7), e05314. https://doi.org/10.2903/j.efsa.2018.5314
Madau, F. A., Arru, B., Furesi, R., & Pulina, P. (2020). Insect farming for feed and food production from a circular business model perspective. Sustainability, 12(13), 5418. https://doi.org/10.3390/su12135418
Manceron, S., Ben Ari, T., & Dumas, P. (2014). Feeding proteins to livestock: Global land use and food vs. feed competition. Oilseeds and fats, Crops and Lipids, 21(4), D408. https://doi.org/10.1051/ocl/2014020
Kim, S. W., Less, J. F., Wang, L., Yan, T., Kiron, V., Kaushik, S. J., & Lei, X. G. (2019). Meeting global feed protein demand: challenge, opportunity, and strategy. Annual Review of Animal Biosciences, 7, 221-243. https://doi.org/10.1146/annurev-animal-030117-014838
van Huis, A., Rumpold, B. A., Van der Fels-Klerx, H. J., & Tomberlin, J. K. (2021). Advancing edible insects as food and feed in a circular economy. Journal of Insects as Food and Feed, 7(5), 935-948. https://doi.org/10.3920/JIFF2021.x005
Winkler, K., Fuchs, R., Rounsevell, M., & Herold, M. (2021). Global land use changes are four times greater than previously estimated. Nature Communications, 12(1), 1-10. https://doi.org/10.1038/s41467-021-22702-2
Song, X. P., Hansen, M. C., Potapov, P., Adusei, B., Pickering, J., Adami, M., Lima, A., Zalles, V., Stehman, S. V., Di Bella, C. M., Conde, M. C., Copati, E. J., Fernandes, L. B., Hernandez-Serna, A., Jantz, S. M., Pickens, A. H., Turubanova, S., & Tyukavina, A. (2021). Massive soybean expansion in South America since 2000 and implications for conservation. Nature Sustainability, 4(9), 784-792. https://doi.org/10.1038/s41893-021-00729-z
Kastens, J. H., Brown, J. C., Coutinho, A. C., Bishop, C. R., & Esquerdo, J. C. D. (2017). Soy moratorium impacts on soybean and deforestation dynamics in Mato Grosso, Brazil. PloS one, 12(4), e0176168. https://doi.org/10.1371/journal.pone.0176168
Lathuilliere, M. J., Miranda, E. J., Bulle, C., Couto, E. G., & Johnson, M. S. (2017). Land occupation and transformation impacts of soybean production in Southern Amazonia, Brazil. Journal of Cleaner Production, 149, 680-689. https://doi.org/10.1016/j.jclepro.2017.02.120
Paiva, P. F. P. R., de Lourdes Pinheiro Ruivo, M., da Silva Júnior, O. M., de Nazaré Martins Maciel, M., Braga, T. G. M., de Andrade, M. M. N., dos Santos Junior, P. C., da Rocha, E. S., de Freitas, T. P. M., da Silva Leite, T. V., Gama, L. H. O. M., de Sousa Santos, L., da Silva, M. G., Silva, E. R. R., & Ferreira, B. M. (2020). Deforestation in protect areas in the Amazon: a threat to biodiversity. Biodiversity and Conservation, 29(1), 19-38. https://doi.org/10.1007/s10531-019-01867-9
Cordeiro, M. R., Rotz, A., Kroebel, R., Beauchemin, K. A., Hunt, D., Bittman, S., Koenig, K. M., & McKenzie, D. B. (2019). Prospects of forage production in northern regions under climate and land-use changes: a case-study of a dairy farm in Newfoundland, Canada. Agronomy, 9(1), 31. https://doi.org/10.3390/agronomy9010031
Alig, R. J., & Ahearn, M. C. (2017). Effects of policy and technological change on land use. In Economics of Rural Land-use Change (pp. 43-56). Routledge. eBook ISBN: 9781315257020
Øverland, M., Mydland, L. T., & Skrede, A. (2019). Marine macroalgae as sources of protein and bioactive compounds in feed for monogastric animals. Journal of the Science of Food and Agriculture, 99(1), 13-24. https://doi.org/10.1002/jsfa.9143
Koesling, M., Kvadsheim, N. P., Halfdanarson, J., Emblemsvåg, J., & Rebours, C. (2021). Environmental impacts of protein-production from farmed seaweed: comparison of possible scenarios in Norway. Journal of Cleaner Production, 307, 127301. https://doi.org/10.1016/j.jclepro.2021.127301
Food and Agriculture Organization of the United Nations. (2013). Food wastage footprint: Impacts on natural resources: Summary report. FAO. Available at https://www.fao.org/3/i3347e/i3347e.pdf
Tonini, D., Albizzati, P. F., & Astrup, T. F. (2018). Environmental impacts of food waste: Learnings and challenges from a case study on UK. Waste Management, 76, 744-766. https://doi.org/10.1016/j.wasman.2018.03.032
Schader, C., Muller, A., Scialabba, N. E. H., Hecht, J., Isensee, A., Erb, K. H., Smith, P., Makkar, H. P. S., Klocke, P., Leiber, F., Schwegler, P., Stolze, M., & Niggli, U. (2015). Impacts of feeding less food-competing feedstuffs to livestock on global food system sustainability. Journal of the Royal Society Interface, 12(113), 20150891. https://doi.org/10.1098/rsif.2015.0891
Doi, H., & Mulia, R. N. (2021). Future Land Use for Insect Meat Production Among Countries: A Global Classification. Frontiers in Nutrition, 8, 661056. https://doi.org/10.3389/fnut.2021.661056
Shah, F., & Wu, W. (2019). Soil and crop management strategies to ensure higher crop productivity within sustainable environments. Sustainability, 11(5), 1485. https://doi.org/10.3390/su11051485
Johnson, M. G. (2018). The role of soil management in sequestering soil carbon. In Soil Management and Greenhouse Effect (pp. 351-364). CRC Press.
Olsson, L., Barbosa, H., Bhadwal, S., Cowie, A., Delusca, K., Flores-Renteria, D., Hermans, K., Jobbagy, E., Kurz, W., Li, D., Sonwa, D. J., Stringer, L. (2019). Land Degradation. In: Climate Change and Land: an IPCC special report on climate change, desertification, land degradation, sustainable land management, food security, and greenhouse gas fluxes in terrestrial ecosystems [Shukla, P. R., Skea, J., Calvo Buendia, E., Masson-Delmotte, V., Pörtner, H. O., Roberts, D. C., Zhai, P., Slade, R., Connors, S., van Diemen, R., Ferrat, M., Haughey, E., Luz, S., Neogi, S., Pathak, M., Petzold, J., Portugal Pereira, J., Vyas, P., Huntley, E., Kissick, K., Belkacemi, M., & Malley, J. (eds.)]
Castanheira, É. G., & Freire, F. (2013). Greenhouse gas assessment of soybean production: implications of land use change and different cultivation systems. Journal of Cleaner Production, 54, 49-60. https://doi.org/10.1016/j.jclepro.2013.05.026
Hoang, N. T., & Kanemoto, K. (2021). Mapping the deforestation footprint of nations reveals growing threat to tropical forests. Nature Ecology & Evolution, 5(6), 845-853. https://doi.org/10.1038/s41559-021-01417-z
Tang, K. H. D., & Yap, P. S. (2020). A Systematic Review of Slash-and-Burn Agriculture as an Obstacle to Future-Proofing Climate Change. In Proceedings of The International Conference on Climate Change (Vol. 4, No. 1). https://doi.org/10.17501/2513258X.2020.4101
Hoffman, E., Cavigelli, M. A., Camargo, G., Ryan, M., Ackroyd, V. J., Richard, T. L., & Mirsky, S. (2018). Energy use and greenhouse gas emissions in organic and conventional grain crop production: accounting for nutrient inflows. Agricultural Systems, 162, 89-96. https://doi.org/10.1016/j.agsy.2018.01.021
Brookes, G., & Barfoot, P. (2020). GM crops: global socio-economic and environmental impacts 1996–2018. Available at: https://www.pgeconomics.co.uk/pdf/globalimpactfinalreportJuly2020.pdf. Accessed on 15 December 2021
Chen, P., Zhu, G., Kim, H. J., Brown, P. B., & Huang, J. Y. (2020). Comparative life cycle assessment of aquaponics and hydroponics in the Midwestern United States. Journal of Cleaner Production, 275, 122888. https://doi.org/10.1016/j.jclepro.2020.122888
Taelman, S. E., De Meester, S., Van Dijk, W., Da Silva, V., & Dewulf, J. (2015). Environmental sustainability analysis of a protein-rich livestock feed ingredient in The Netherlands: Microalgae production versus soybean import. Resources, Conservation and Recycling, 101, 61-72. https://doi.org/10.1016/j.resconrec.2015.05.013
Vandermeersch, T., Alvarenga, R. A. F., Ragaert, P., & Dewulf, J. (2014). Environmental sustainability assessment of food waste valorization options. Resources, Conservation and Recycling, 87, 57-64. https://doi.org/10.1016/j.resconrec.2014.03.008
van Zanten, H. H., Mollenhorst, H., Oonincx, D. G., Bikker, P., Meerburg, B. G., & de Boer, I. J. (2015). From environmental nuisance to environmental opportunity: housefly larvae convert waste to livestock feed. Journal of Cleaner Production, 102, 362-369. https://doi.org/10.1016/j.jclepro.2015.04.106
Wang, Y. S., & Shelomi, M. (2017). Review of black soldier fly (Hermetia illucens) as animal feed and human food. Foods, 6(10), 91. https://doi.org/10.3390/foods6100091
Bosch, G., Van Zanten, H. H. E., Zamprogna, A., Veenenbos, M., Meijer, N. P., Van der Fels-Klerx, H. J., & Van Loon, J. J. A. (2019). Conversion of organic resources by black soldier fly larvae: legislation, efficiency and environmental impact. Journal of Cleaner Production, 222, 355-363. https://doi.org/10.1016/j.jclepro.2019.02.270
Jansson, A., & Berggren, Å. (2015). Insects as food-something for the future? A report from Future Agriculture. Uppsala, Swedish University of Agricultural Sciences (SLU) Available at https://pub.epsilon.slu.se/12935/7/jansson_a_berggren_a_151230.pdf
Lima, M., da Silva Junior, C. A., Rausch, L., Gibbs, H. K., & Johann, J. A. (2019). Demystifying sustainable soy in Brazil. Land Use Policy, 82, 349-352. https://doi.org/10.1016/j.landusepol.2018.12.016
Pacheco, F. A. L., Fernandes, L. F. S., Junior, R. F. V., Valera, C. A., & Pissarra, T. C. T. (2018). Land degradation: Multiple environmental consequences and routes to neutrality. Current Opinion in Environmental Science & Health, 5, 79-86. https://doi.org/10.1016/j.coesh.2018.07.002
Andow, D. A. (2003). UK farm-scale evaluations of transgenic herbicide-tolerant crops. Nature Biotechnology, 21(12), 1453-1454.
Tsatsakis, A. M., Nawaz, M. A., Kouretas, D., Balias, G., Savolainen, K., Tutelyan, V. A., Golokhvast, K. S., Lee, J. D., Yang, S. H., & Chung, G. (2017). Environmental impacts of genetically modified plants: a review. Environmental Research, 156, 818-833. http://dx.doi.org/10.1016/j.envres.2017.03.011
Schütte, G., Eckerstorfer, M., Rastelli, V., Reichenbecher, W., Restrepo-Vassalli, S., Ruohonen-Lehto, M., Wuest Saucy, A. G., & Mertens, M. (2017). Herbicide resistance and biodiversity: agronomic and environmental aspects of genetically modified herbicide-resistant plants. Environmental Sciences Europe, 29(1), 1-12. https://doi.org/10.1186/s12302-016-0100-y
Relyea, R. A. (2005). The impact of insecticides and herbicides on the biodiversity and productivity of aquatic communities. Ecological applications, 15(2), 618-627. https://doi.org/10.1890/03-5342
Food and Agriculture Organization (FAO). (2016a). Environmental performance of animal feeds supply chains: Guidelines for assessment. Livestock Environmental Assessment and Performance Partnership. FAO, Rome, Italy
Zortea, R. B., Maciel, V. G., & Passuello, A. (2018). Sustainability assessment of soybean production in Southern Brazil: A life cycle approach. Sustainable Production and Consumption, 13, 102-112. https://doi.org/10.1016/j.spc.2017.11.002
Paul, M. J., Nuccio, M. L., & Basu, S. S. (2018). Are GM crops for yield and resilience possible?. Trends in Plant Science, 23(1), 10-16. https://doi.org/10.1016/j.tplants.2017.09.007
Zheng, Y., Jin, R., Zhang, X., Wang, Q., & Wu, J. (2019). The considerable environmental benefits of seaweed aquaculture in China. Stochastic Environmental Research and Risk Assessment, 33(4), 1203-1221. https://doi.org/10.1007/s00477-019-01685-z
Gao, G., Gao, L., Jiang, M., Jian, A., & He, L. (2021). The potential of seaweed cultivation to achieve carbon neutrality and mitigate deoxygenation and eutrophication. Environmental Research Letters, 17(1), 014018. https://doi.org/10.1088/1748-9326/ac3fd9
Salemdeeb, R., Zu Ermgassen, E. K., Kim, M. H., Balmford, A., & Al-Tabbaa, A. (2017). Environmental and health impacts of using food waste as animal feed: a comparative analysis of food waste management options. Journal of Cleaner Production, 140, 871-880. https://doi.org/10.1016/j.jclepro.2016.05.049
Trabue, S. L., Kerr, B. J., Scoggin, K. D., Andersen, D., & Van Weelden, M. (2021). Swine diets impact manure characteristics and gas emissions: Part II protein source. Science of The Total Environment, 763, 144207.
Elahi, U., Xu, C., Wang, J., Lin, J., Wu, S., Zhang, H., & Qi, G. (2022). Insect meal as a feed ingredient for poultry. Animal Bioscience, 35(2), 332-346. https://doi.org/10.5713/ab.21.0435
Huygens, D., Orveillon, G., Lugato, E., Tavazzi, S., Comero, S., Jones, A., Gawlik, B., & Saveyn, H. (2020). Technical proposals for the safe use of processed manure above the threshold established for Nitrate Vulnerable Zones by the Nitrates Directive (91/676/EEC). Publications Office of the European Union, Luxembourg.
Mekonnen, M. M., & Hoekstra, A. Y. (2020). Sustainability of the blue water footprint of crops. Advances in Water Resources, 143, 103679. https://doi.org/10.1016/j.advwatres.2020.103679
Mekonnen, M. M., & Hoekstra, A. Y. (2011). The green, blue and grey water footprint of crops and derived crop products. Hydrology and Earth System Sciences, 15(5), 1577-1600. https://doi.org/10.5194/hess-15-1577-2011
Dinar, A., Tieu, A., & Huynh, H. (2019). Water scarcity impacts on global food production. Global Food Security, 23, 212-226. https://doi.org/10.1016/j.gfs.2019.07.007
Kumar, K., Gambhir, G., Dass, A., Tripathi, A. K., Singh, A., Jha, A. K., Yadava, P., Choudhary, M., & Rakshit, S. (2020). Genetically modified crops: current status and future prospects. Planta, 251(91). https://doi.org/10.1007/s00425-020-03372-8
Atzori, G., Nissim, W. G., Caparrotta, S., Santantoni, F., & Masi, E. (2019). Seawater and water footprint in different cropping systems: a chicory (Cichorium intybus L.) case study. Agricultural Water Management, 211, 172-177. https://doi.org/10.1016/j.agwat.2018.09.040
Cifuentes‐Torres, L., Mendoza‐Espinosa, L. G., Correa‐Reyes, G., & Daesslé, L. W. (2021). Hydroponics with wastewater: a review of trends and opportunities. Water and Environment Journal, 35(1), 166-180. https://doi.org/10.1111/wej.12617
Campbell, I., Macleod, A., Sahlmann, C., Neves, L., Funderud, J., Øverland, M., Hughes, A. D., & Stanley, M. (2019). The environmental risks associated with the development of seaweed farming in Europe-prioritizing key knowledge gaps. Frontiers in Marine Science, 6, 107. https://doi.org/10.3389/fmars.2019.00107
Santos, J. F. S., & Naval, L. P. (2020). Spatial and temporal dynamics of water footprint for soybean production in areas of recent agricultural expansion of the Brazilian savannah (Cerrado). Journal of Cleaner Production, 251, 119482. https://doi.org/10.1016/j.jclepro.2019.119482
Zhao, X., Liao, X., Chen, B., Tillotson, M. R., Guo, W., & Li, Y. (2019). Accounting global grey water footprint from both consumption and production perspectives. Journal of Cleaner Production, 225, 963-971. https://doi.org/10.1016/j.jclepro.2019.04.037
Malone, T. C., & Newton, A. (2020). The globalization of cultural eutrophication in the coastal ocean: causes and consequences. Frontiers in Marine Science, 7, 670. https://doi.org/10.3389/fmars.2020.00670
Food and Agriculture Organization (FAO). (2016b). Handbook on agricultural cost of production statistics: Guidelines for data collection, compilation and dissemination. FAO, Rome, Italy.
Oliveira, A. L. R. D., Filassi, M., Lopes, B. F. R., & Marsola, K. B. (2020). Logistical transportation routes optimization for Brazilian soybean: an application of the origin-destination matrix. Ciência Rural, 51. http://doi.org/10.1590/0103-8478cr20190786
Lo, B., Kasapis, S., & Farahnaky, A. (2021). Lupin protein: Isolation and techno-functional properties, a review. Food Hydrocolloids, 112, 106318. https://doi.org/10.1016/j.foodhyd.2020.106318
Popp, J., Harangi-Rákos, M., Gabnai, Z., Balogh, P., Antal, G., & Bai, A. (2016). Biofuels and their co-products as livestock feed: global economic and environmental implications. Molecules, 21(3), 285. https://doi.org/10.3390/molecules21030285
O’Malley, J., & Searle, S. (2021). “The Impact of the US Renewable Fuel Standard on Food and Feed Prices”. Technical report, International Council on Clean Transportation. https://theicct.org/sites/default/files/publications/RFS-and-feed-prices-jan2021.pdf
van den Burg, S. W., van Duijn, A. P., Bartelings, H., van Krimpen, M. M., & Poelman, M. (2016). The economic feasibility of seaweed production in the North Sea. Aquaculture Economics & Management, 20(3), 235-252. https://doi.org/10.1080/13657305.2016.1177859
Emblemsvåg, J., Kvadsheim, N. P., Halfdanarson, J., Koesling, M., Nystrand, B. T., Sunde, J., & Rebours, C. (2020). Strategic considerations for establishing a large-scale seaweed industry based on fish feed application: a Norwegian case study. Journal of Applied Phycology, 32(6), 4159-4169. https://doi.org/10.1007/s10811-020-02234-w
Taghizadeh-Hesary, F., Rasoulinezhad, E., & Yoshino, N. (2019). Energy and food security: Linkages through price volatility. Energy Policy, 128, 796-806. https://doi.org/10.1016/j.enpol.2018.12.043
Punzi, M. T. (2019). The impact of energy price uncertainty on macroeconomic variables. Energy Policy, 129, 1306-1319. https://doi.org/10.1016/j.enpol.2019.03.015
Girma, F., & Gebremariam, B. (2018). Review on hydroponic feed value to livestock production. Journal of Scientific and Innovative Research, 7(4), 106-109.
Greenfeld, A., Becker, N., McIlwain, J., Fotedar, R., & Bornman, J. F. (2019). Economically viable aquaponics? Identifying the gap between potential and current uncertainties. Reviews in Aquaculture, 11(3), 848-862. https://doi.org/10.1111/raq.12269
Goddek, S., Delaide, B., Mankasingh, U., Ragnarsdottir, K. V., Jijakli, H., & Thorarinsdottir, R. (2015). Challenges of sustainable and commercial aquaponics. Sustainability, 7(4), 4199-4224. https://doi.org/10.3390/su7044199
Palm, H. W., Knaus, U., Appelbaum, S., Goddek, S., Strauch, S. M., Vermeulen, T., Jijakli, M. H., & Kotzen, B. (2018). Towards commercial aquaponics: a review of systems, designs, scales and nomenclature. Aquaculture International, 26(3), 813-842. https://doi.org/10.1007/s10499-018-0249-z
Arru, B., Furesi, R., Gasco, L., Madau, F. A., & Pulina, P. (2019). The introduction of insect meal into fish diet: The first economic analysis on European sea bass farming. Sustainability, 11(6), 1697. https://doi.org/10.3390/su11061697
WWF . (2021). The future of feed: a WWF roadmap to accelerating insect protein in UK feeds. Available at: https://www.wwf.org.uk/sites/default/files/2021-06/The_future_of_feed_July_2021.pdf. Accessed on 15 December 2021
Chia, S. Y., Tanga, C. M., van Loon, J. J., & Dicke, M. (2019). Insects for sustainable animal feed: Inclusive business models involving smallholder farmers. Current Opinion in Environmental Sustainability, 41, 23-30. https://doi.org/10.1016/j.cosust.2019.09.003
Oonincx, D. G. A. B., & Finke, M. D. (2021). Nutritional value of insects and ways to manipulate their composition. Journal of Insects as Food and Feed, 7(5), 639-659. https://doi.org/10.3920/JIFF2020.0050
Pinotti, L., & Ottoboni, M. (2021). Substrate as insect feed for bio-mass production. Journal of Insects as Food and Feed, 7(5), 585-596. https://doi.org/10.3920/JIFF2020.0110
de Miranda, J. R., Granberg, F., Onorati, P., Jansson, A., & Berggren, Å. (2021). Virus prospecting in crickets—Discovery and strain divergence of a novel iflavirus in wild and cultivated Acheta domesticus. Viruses, 13(3), 364. https://doi.org/10.3390/v13030364
Truzzi, C., Annibaldi, A., Girolametti, F., Giovannini, L., Riolo, P., Ruschioni, S., Olivotto, I., & Illuminati, S. (2020). A chemically safe way to produce insect biomass for possible application in feed and food production. International journal of environmental research and public health, 17(6), 2121. https://doi.org/10.3390/ijerph17062121
Ojha, S., Bußler, S., Psarianos, M., Rossi, G., & Schlüter, O. K. (2021). Edible insect processing pathways and implementation of emerging technologies. Journal of Insects as Food and Feed, 7(5), 877-900. https://doi.org/10.3920/JIFF2020.0121
Sindermann, D., Heidhues, J., Kirchner, S., Stadermann, N., & Kühl, A. (2021). Industrial processing technologies for insect larvae. Journal of Insects as Food and Feed, 7(5), 857-875. https://doi.org/10.3920/JIFF2020.0103
Lioutas, E. D., & Charatsari, C. (2021). Enhancing the ability of agriculture to cope with major crises or disasters: What the experience of COVID-19 teaches us. Agricultural Systems, 187, 103023. https://doi.org/10.1016/j.agsy.2020.103023
Rzymski, P., Kulus, M., Jankowski, M., Dompe, C., Bryl, R., Petitte, J. N., Kempisty, B., & Mozdziak, P. (2021). COVID-19 pandemic is a call to search for alternative protein sources as food and feed: A review of possibilities. Nutrients, 13(1), 150. https://doi.org/10.3390/nu13010150
Henry, R. (2020). Innovations in agriculture and food supply in response to the COVID-19 pandemic. Molecular Plant, 13(8), 1095. https://doi.org/10.1016/j.molp.2020.07.011
Choi, H. S., Jansson, T., Matthews, A., & Mittenzwei, K. (2021). European agriculture after Brexit: does anyone benefit from the divorce?. Journal of Agricultural Economics, 72(1), 3-24. https://doi.org/10.1111/1477-9552.12396
Yao, G., Zhang, X., Davidson, E. A., & Taheripour, F. (2021). The increasing global environmental consequences of a weakening US–China crop trade relationship. Nature Food, 2(8), 578-586. https://doi.org/10.1038/s43016-021-00338-1
Institute Du Porc (IFIP). (2022). Crise Russo-Ukrainienne : impacts sur les marchés des matières premières pour les filières animals. Available at: https://ifip.asso.fr/crise-russo-ukrainienne-impacts-sur-les-marches-des-matieres-premieres-pour-les-filieres-animales/. Accessed on 1 March 2022.
Food and Agriculture Organization Statistics Division (FAOSTAT). (2021). Available at: https://www.fao.org/faostat/en/#home. Accessed on 1 March 2022.
Gasco, L., Biasato, I., Dabbou, S., Schiavone, A., & Gai, F. (2019). Animals fed insect-based diets: State-of-the-art on digestibility, performance and product quality. Animals, 9(4), 170. https://doi.org/10.3390/ani9040170
Gasco, L., Finke, M., & Van Huis, A. (2018). Can diets containing insects promote animal health?. Journal of Insects as Food and Feed, 4(1), 1-4. https://doi.org/10.3920/JIFF2018.x001
Edwards 3rd, H. M., Douglas, M. W., Parsons, C. M., & Baker, D. H. (2000). Protein and energy evaluation of soybean meals processed from genetically modified high-protein soybeans. Poultry Science, 79(4), 525-527. https://doi.org/10.1093/ps/79.4.525
Sauvant, D., Perez, J. M., & Tran, G. (Eds.). (2004). Tables of composition and nutritional value of feed materials: pigs, poultry, cattle, sheep, goats, rabbits, horses and fish. Wageningen Academic Publishers.
Giraldo, P. A., Shinozuka, H., Spangenberg, G. C., Cogan, N. O., & Smith, K. F. (2019). Safety assessment of genetically modified feed: is there any difference from food?. Frontiers in Plant Science, 10, 1592. https://doi.org/10.3389/fpls.2019.01592
Coudert, E., Baéza, E., & Berri, C. (2020). Use of algae in poultry production: A review. World's Poultry Science Journal, 76(4), 767-786. https://doi.org/10.1080/00439339.2020.1830012
Morais, T., Inácio, A., Coutinho, T., Ministro, M., Cotas, J., Pereira, L., & Bahcevandziev, K. (2020). Seaweed potential in the animal feed: A review. Journal of Marine Science and Engineering, 8(8), 559. https://doi.org/10.3390/jmse8080559
Sońta, M., Rekiel, A., Więcek, J., Batorska, M., & Puppel, K. (2021). Alternative protein sources vs. GM soybean meal as feedstuff for pigs-meat quality and health-promoting indicators. Animals, 11(1), 177. https://doi.org/10.3390/ani11010177
EFSA Panel on Genetically Modified Organisms (GMO), Naegeli, H., Bresson, J. L., Dalmay, T., Dewhurst, I. C., Epstein, M. M., Firbank, L. G., Guerche, P., Hejatko, J., Moreno, F. J., Mullins, E., Nogué, F., Rostoks, N., Serrano, J. J. S., Savoini, G., Veromann, E., & Veronesi, F. (2020). Assessment of genetically modified soybean SYHT 0H2 for food and feed uses, import and processing, under Regulation (EC) No 1829/2003 (application EFSA‐GMO‐DE‐2012‐111). EFSA Journal, 18(1), e05946.
Buzoianu, S. G., Walsh, M. C., Rea, M. C., Cassidy, J. P., Ryan, T. P., Ross, R. P., Gardiner, G. E., & Lawlor, P. G. (2013). Transgenerational effects of feeding genetically modified maize to nulliparous sows and offspring on offspring growth and health. Journal of Animal Science, 91(1), 318-330. https://doi.org/10.2527/jas.2012-5360
Kebede, H., Liu, X., Jin, J., & Xing, F. (2020). Current status of major mycotoxins contamination in food and feed in Africa. Food Control, 110, 106975. https://doi.org/10.1016/j.foodcont.2019.106975
Conte, G., Fontanelli, M., Galli, F., Cotrozzi, L., Pagni, L., & Pellegrini, E. (2020). Mycotoxins in feed and food and the role of ozone in their detoxification and degradation: An update. Toxins, 12(8), 486. https://doi.org/10.3390/toxins12080486
Mahato, D. K., Devi, S., Pandhi, S., Sharma, B., Maurya, K. K., Mishra, S., Dhawan, K., Selvakumar, R., Kamle, M., Mishra, A. K., & Kumar, P. (2021). Occurrence, impact on agriculture, human health, and management strategies of zearalenone in food and feed: A review. Toxins, 13(2), 92. https://doi.org/10.3390/toxins13020092
Alshannaq, A. F., Gibbons, J. G., Lee, M. K., Han, K. H., Hong, S. B., & Yu, J. H. (2018). Controlling aflatoxin contamination and propagation of Aspergillus flavus by a soy-fermenting Aspergillus oryzae strain. Scientific Reports, 8(1), 1-14. https://doi.org/10.1038/s41598-018-35246-1
Netherwood, T., Martín-Orúe, S. M., O'Donnell, A. G., Gockling, S., Graham, J., Mathers, J. C., & Gilbert, H. J. (2004). Assessing the survival of transgenic plant DNA in the human gastrointestinal tract. Nature Biotechnology, 22(2), 204-209. https://doi.org/10.1038/nbt934
Dona, A., & Arvanitoyannis, I. S. (2009). Health risks of genetically modified foods. Critical Reviews in Food Science and Nutrition, 49(2), 164-175. https://doi.org/10.1080/10408390701855993
Korwin-Kossakowska, A., Gralak, B., Faliszewska, G., & Karpiniak, E. (2020). The influence of GMO feed on ecosystem stability of the gastrointestinal tract in different species-a review. Animal Science Papers & Reports, 38(3).
Diaz-Llano, G., & Smith, T. K. (2006). Effects of feeding grains naturally contaminated with Fusarium mycotoxins with and without a polymeric glucomannan mycotoxin adsorbent on reproductive performance and serum chemistry of pregnant gilts. Journal of Animal Science, 84(9), 2361-2366. https://doi.org/10.2527/jas.2006-213
Dunn, E. S., Vicini, J. L., Glenn, K. C., Fleischer, D. M., & Greenhawt, M. J. (2017). The allergenicity of genetically modified foods from genetically engineered crops: A narrative and systematic review. Annals of Allergy and Asthma Immunology, 119(3), 2124-222.e3.
Dubois, A. E., Pagliarani, G., Brouwer, R. M., Kollen, B. J., Dragsted, L. O., Eriksen, F. D., Callesen, O., Gilissen, L. J. W. J., Krens, F. A., Visser, R. G. F., Smulders, M. J. M., Vlieg-Boerstra, B. J., Flokstra-de Blok, B. J., & Van De Weg, W. E. (2015). First successful reduction of clinical allergenicity of food by genetic modification: Mal d 1‐silenced apples cause fewer allergy symptoms than the wild‐type cultivar. Allergy, 70(11), 1406-1412. https://doi.org/10.1111/all.12684
Becton, L., Davis, P., Sundberg, P., & Wilkinson, L. (2022). Feed safety collaborations: Experiences, progress and challenges. Transboundary and Emerging Diseases, 69(1), 182-188. https://doi.org/10.1111/tbed.14297
Ráduly, Z., Price, R. G., Dockrell, M. E., Csernoch, L., & Pócsi, I. (2021). Urinary Biomarkers of Mycotoxin Induced Nephrotoxicity—Current Status and Expected Future Trends. Toxins, 13(12), 848. https://doi.org/10.3390/toxins13120848
Magnoli, A. P., Poloni, V. L., & Cavaglieri, L. (2019). Impact of mycotoxin contamination in the animal feed industry. Current Opinion in Food Science, 29, 99-108. https://doi.org/10.1016/j.cofs.2019.08.009
Santo, R. E., Kim, B. F., Goldman, S. E., Dutkiewicz, J., Biehl, E., Bloem, M. W., Neff, R. A., & Nachman, K. E. (2020). Considering plant-based meat substitutes and cell-based meats: a public health and food systems perspective. Frontiers in Sustainable Food Systems, 134. https://doi.org/10.3389/fsufs.2020.00134
Pinotti, L., Giromini, C., Ottoboni, M., Tretola, M., & Marchis, D. (2019). Insects and former foodstuffs for upgrading food waste biomasses/streams to feed ingredients for farm animals. Animal, 13(7), 1365-1375. https://doi.org/10.1017/S1751731118003622
Schrögel, P., & Wätjen, W. (2019). Insects for food and feed-safety aspects related to mycotoxins and metals. Foods, 8(8), 288. https://doi.org/10.3390/foods8080288
Smith, M., Love, D. C., Rochman, C. M., & Neff, R. A. (2018). Microplastics in seafood and the implications for human health. Current Environmental Health Reports, 5(3), 375-386. https://doi.org/10.1007/s40572-018-0206-z
Prata, J. C., da Costa, J. P., Lopes, I., Duarte, A. C., & Rocha-Santos, T. (2020). Environmental exposure to microplastics: An overview on possible human health effects. Science of the Total Environment, 702, 134455. https://doi.org/10.1016/j.scitotenv.2019.134455
Krasucka, P., Bogusz, A., Baranowska-Wójcik, E., Czech, B., Szwajgier, D., Rek, M., Ok, Y. S., & Oleszczuk, P. (2022). Digestion of plastics using in vitro human gastrointestinal tract and their potential to adsorb emerging organic pollutants. Science of The Total Environment, 843, 157108. https://doi.org/10.1016/j.scitotenv.2022.157108
Advisory Committee on Animal Feedingstuffs (ACAF) (2009). Potential for carry-over of allergens from animal feed into derived animal products. Available at: https://acaf.food.gov.uk/sites/default/files/mnt/drupal_data/sources/files/multimedia/pdfs/committee/acaf0904.pdf Accessed on 20 March 2022
Testa, M., Stillo, M., Maffei, G., Andriolo, V., Gardois, P., & Zotti, C. M. (2017). Ugly but tasty: A systematic review of possible human and animal health risks related to entomophagy. Critical Reviews in Food Science and Nutrition, 57(17), 3747-3759. https://doi.org/10.1080/10408398.2016.1162766
Bingemann, T. A., Santos, C. B., Russell, A. F., & Anagnostou, A. (2019). Lupin: An emerging food allergen in the United States. Annals of Allergy, Asthma & Immunology, 122(1), 8-10. https://doi.org/10.1016/j.anai.2018.09.467
Gultekin, F., Oner, M. E., Savas, H. B., & Dogan, B. (2020). Food additives and microbiota. Northern clinics of Istanbul, 7(2), 192. https://doi.org/10.14744/nci.2019.92499
Rinninella, E., Cintoni, M., Raoul, P., Gasbarrini, A., & Mele, M. C. (2020). Food additives, gut microbiota, and irritable Bowel syndrome: A hidden track. International Journal of Environmental Research and Public Health, 17(23), 8816. https://doi.org/10.3390/ijerph17238816
Saito, Y., Saito, H., & Sembokuya, Y. (2009). Consumer evaluations of pork from hogs raised on recycled food waste. Agricultural Information Research, 18(3), 152-161. https://doi.org/10.3173/air.18.152
Borrello, M., Caracciolo, F., Lombardi, A., Pascucci, S., & Cembalo, L. (2017). Consumers’ perspective on circular economy strategy for reducing food waste. Sustainability, 9(1), 141. https://doi.org/10.3390/su9010141
Bhatt, S., Lee, J., Deutsch, J., Ayaz, H., Fulton, B., & Suri, R. (2018). From food waste to value‐added surplus products (VASP): Consumer acceptance of a novel food product category. Journal of Consumer Behaviour, 17(1), 57-63. https://doi.org/10.1002/cb.1689
Mens, A., Cone, J., van den Borne, B., & Bosch, G. (2021). Capacities of animals to make agri-food systems more circular (No. 1323). Wageningen Livestock Research. Public Report 1323. Available at https://library.wur.nl/WebQuery/wurpubs/fulltext/548324
Westendorf, M. L. (Ed.). (2000). Food waste to animal feed (1st ed.). Ames: Iowa State University Press
Jayathilake, N., Aheeyar, M., & Drechsel, P. (2022). Food Waste to Livestock Feed: Prospects and Challenges for Swine Farming in Peri-urban Sri Lanka. Circular Economy and Sustainability, 1-15. https://doi.org/10.1007/s43615-022-00168-8
Swain, B. B., & Teufel, N. (2017). The impact of urbanisation on crop–livestock farming system: a comparative case study of India and Bangladesh. Journal of Social and Economic Development, 19(1), 161-180. https://doi.org/10.1007/s40847-017-0038-y
Marinoudi, V., Sørensen, C. G., Pearson, S., & Bochtis, D. (2019). Robotics and labour in agriculture. A context consideration. Biosystems Engineering, 184, 111-121. https://doi.org/10.1016/j.biosystemseng.2019.06.013
Elahi, E., Weijun, C., Zhang, H., & Abid, M. (2019). Use of artificial neural networks to rescue agrochemical-based health hazards: A resource optimisation method for cleaner crop production. Journal of Cleaner Production, 238, 117900. https://doi.org/10.1016/j.jclepro.2019.117900
Rukundo, E., Liu, S., Dong, Y., Rutebuka, E., Asamoah, E. F., Xu, J., & Wu, X. (2018). Spatio-temporal dynamics of critical ecosystem services in response to agricultural expansion in Rwanda, East Africa. Ecological Indicators, 89, 696-705. https://doi.org/10.1016/j.ecolind.2018.02.032
Flach, R., Abrahão, G., Bryant, B., Scarabello, M., Soterroni, A. C., Ramos, F. M., Valin, H., Obersteiner, M., & Cohn, A. S. (2021). Conserving the Cerrado and Amazon biomes of Brazil protects the soy economy from damaging warming. World Development, 146, 105582. https://doi.org/10.1016/j.worlddev.2021.105582
Weindl, I., Ost, M., Wiedmer, P., Schreiner, M., Neugart, S., Klopsch, R., Kühnhold, H., Kloas, W., Henkel, I. M., Schlüter, O., Bußler, S., Bellingrath-Kimura, S. D., Ma, H., Grune, T., Rolinski, S., & Klaus, S. (2020). Sustainable food protein supply reconciling human and ecosystem health: A Leibniz Position. Global Food Security, 25, 100367. https://doi.org/10.1016/j.gfs.2020.100367
Verbeke, W., Spranghers, T., De Clercq, P., De Smet, S., Sas, B., & Eeckhout, M. (2015). Insects in animal feed: Acceptance and its determinants among farmers, agriculture sector stakeholders and citizens. Animal Feed Science and Technology, 204, 72-87. https://doi.org/10.1016/j.anifeedsci.2015.04.001
Onwezen, M. C., Van den Puttelaar, J., Verain, M. C. D., & Veldkamp, T. (2019). Consumer acceptance of insects as food and feed: The relevance of affective factors. Food Quality and Preference, 77, 51-63. https://doi.org/10.1016/j.foodqual.2019.04.011
Altmann, B. A., Anders, S., Risius, A., & Mörlein, D. (2022). Information effects on consumer preferences for alternative animal feedstuffs. Food Policy, 106, 102192. https://doi.org/10.1016/j.foodpol.2021.102192
Khaemba, C. N., Kidoido, M. M., Owuor, G., & Tanga, C. M. (2022). Consumers’ perception towards eggs from laying hens fed commercial black soldier fly (Hermetia illucens) larvae meal-based feeds. Poultry Science, 101(3), 101645. https://doi.org/10.1016/j.psj.2021.101645
Montgomery, H., Haughey, S. A., & Elliott, C. T. (2020). Recent food safety and fraud issues within the dairy supply chain (2015–2019). Global Food Security, 26, 100447. https://doi.org/10.1016/j.gfs.2020.100447
Schiffling, S., & Valantasis Kanellos, N. (2022). Five essential commodities that will be hit by war in Ukraine. The Conversation. https://researchonline.ljmu.ac.uk/id/eprint/16422
United States Department of Agriculture, Foreign Agricultural Service (USDA). (2022). Grain and Feed Update. Available at: https://www.fas.usda.gov/. Accessed on 1 March 2022.
Patterson, J., Schulz, K., Vervoort, J., Van Der Hel, S., Widerberg, O., Adler, C., Hurlbert, M., Anderton, K., Sethi, M., & Barau, A. (2017). Exploring the governance and politics of transformations towards sustainability. Environmental Innovation and Societal Transitions, 24, 1-16. https://doi.org/10.1016/j.eist.2016.09.001
Green, A., Nemecek, T., Chaudhary, A., & Mathys, A. (2020). Assessing nutritional, health, and environmental sustainability dimensions of agri-food production. Global Food Security, 26, 100406. https://doi.org/10.1016/j.gfs.2020.100406
Klapwijk, C. J., Van Wijk, M. T., Rosenstock, T. S., van Asten, P. J., Thornton, P. K., & Giller, K. E. (2014). Analysis of trade-offs in agricultural systems: current status and way forward. Current Opinion in Environmental Sustainability, 6, 110-115. https://doi.org/10.1016/j.cosust.2013.11.012
Kanter, D. R., Musumba, M., Wood, S. L., Palm, C., Antle, J., Balvanera, P., Dale, V. H., Havlik, P., Kline, K. L., Scholes, R. J., Thornton, P., Tittonell, P., & Andelman, S. (2018). Evaluating agricultural trade-offs in the age of sustainable development. Agricultural Systems, 163, 73-88. https://doi.org/10.1016/j.agsy.2016.09.010
Rosenzweig, C. E., Antle, J., & Elliott, J. (2015). Assessing impacts of climate change on food security worldwide. Eos, 97. https://doi.org/10.1029/2016EO047387
Nyström, M., Jouffray, J. B., Norström, A. V., Crona, B., Søgaard Jørgensen, P., Carpenter, S. R., Bodin, Ö., Galaz, V., & Folke, C. (2019). Anatomy and resilience of the global production ecosystem. Nature, 575(7781), 98-108. https://doi.org/10.1038/s41586-019-1712-3
Brick, C., Freeman, A. L., Wooding, S., Skylark, W. J., Marteau, T. M., & Spiegelhalter, D. J. (2018). Winners and losers: communicating the potential impacts of policies. Palgrave Communications, 4(1), 1-13. https://doi.org/10.1057/s41599-018-0121-9
Journeault, M., Perron, A., & Vallières, L. (2021). The collaborative roles of stakeholders in supporting the adoption of sustainability in SMEs. Journal of Environmental Management, 287, 112349. https://doi.org/10.1016/j.jenvman.2021.112349
United Nations Department of Economic and Social Affairs Sustainable Development (UNDESA). (2022). The 17 Sustainable Development Goals. Available at: https://sdgs.un.org/goals. Accessed on 10 February 2022
FAO. (2013). Edible insects: Future prospects for food and feed security. Available at: https://www.fao.org/3/i3253e/i3253e.pdf. Accessed on 10 February 2022
Makkar, H. P., Tran, G., Heuzé, V., & Ankers, P. (2014). State-of-the-art on use of insects as animal feed. Animal Feed Science and Technology, 197, 1-33. https://doi.org/10.1016/j.anifeedsci.2014.07.008
Grant, W. (2016). The challenges facing UK farmers from Brexit. EuroChoices, 15(2), 11-16. https://doi.org/10.1111/1746-692X.12127
Swinbank, A. (2017). World trade rules and the policy options for British agriculture post-Brexit. Briefing paper, 7, 12.
Chang, W. W. (2018). Brexit and its economic consequences. The world economy, 41(9), 2349-2373. https://doi.org/10.1111/twec.12685
Zupaniec, M., Schafft, H. A., Lindemann, A. K., Pieper, R., & Mader, A. (2021). Critical factors for food safety in global commodity flows with a focus on logistics–A case study on Mycotoxin contamination of agri-bulk commodities. Operations and Supply Chain Management: An International Journal, 14(4), 545-563. http://doi.org/10.31387/oscm0470323
Alava, J. J., Cheung, W. W., Ross, P. S., & Sumaila, U. R. (2017). Climate change–contaminant interactions in marine food webs: Toward a conceptual framework. Global Change Biology, 23(10), 3984-4001. https://doi.org/10.1111/gcb.13667
Revision log
Published: 26 April 2023
Last updated: 24 April 2024